Nanoparticles for Chemoimmunotherapy Against Triple-Negative Breast Cancer
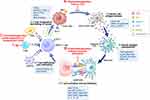
1Department of Breast Surgery, China-Japan Union Hospital of Jilin University, Changchun, People’s Republic of China; 2Department of Scientific Research Center, China-Japan Union Hospital of Jilin University, Changchun, People’s Republic of China; 3Breast Surgery, Jilin Province Tumor Hospital, Changchun, People’s Republic of China
Correspondence: Hua Xing, Department of Breast Surgery, China-Japan Union Hospital of Jilin University, Xian Tai Street, No. 126, Changchun, Jilin, 130033, People’s Republic of China, Tel +86 139 4412 7842, Email [email protected]
Abstract: Triple-negative breast cancer (TNBC) exhibits high recurrence and mortality rates because of the lack of effective treatment targets. Surgery and traditional chemotherapy are the primary treatment options. Immunotherapy shows high potential for treating various cancers but exhibits limited efficacy against TNBC as a monotherapy. Chemoimmunotherapy has broad prospects for applications for cancer treatment conferred through the synergistic immunomodulatory and anti-tumor effects of chemotherapy and immunotherapeutic strategies. However, improving the efficacy of synergistic therapy and reducing the side effects of multiple drugs remain to be the main challenges in chemoimmunotherapy against TNBC. Nanocarriers can target both cancer and immune cells, promote drug accumulation, and show minimal toxicity, making them ideal delivery systems for chemotherapeutic and immunotherapeutic agents. In this review, we introduce the immunomodulatory effects of chemotherapy and combined mechanisms of chemoimmunotherapy, followed by a summary of nanoparticle-mediated chemoimmunotherapeutic strategies used for treating TNBC. This up-to-date synthesis of relevant findings in the field merits contemplation, while considering avenues of investigation to enable advances in the field.
Keywords: chemotherapy, drug-delivery system, immunotherapy, mechanism, targeted delivery
Introduction
Triple-negative breast cancer (TNBC), which lacks expression of the estrogen receptor, progesterone receptor, and human epidermal growth factor receptor 2, accounts for 15–20% of all breast cancer cases.1,2 Anthracycline- and taxane-based chemotherapy are the main treatments for TNBC. However, 30–40% of patients with early TNBC develop metastatic disease and die from this cancer.3 Recent clinical trials showed that progress has been made in using immunotherapy to treat TNBC. Compared with other breast cancer subtypes, TNBC exhibits high immunogenicity manifested as high enrichment of tumor-infiltrating lymphocytes (TILs) and increased expression levels of programmed cell death ligand 1 (PD-L1); therefore, patients with TNBC are more likely to benefit from immunotherapy.4 Moreover, clinical data revealed that chemotherapy combined with immune checkpoint inhibitors (ICIs) can significantly improve the progression-free survival and overall survival of patients with TNBC; PD-L1-positive subgroup showing benefits from combination treatment.5,6 Therefore, the Food and Drug Administration (FDA) approved atezolizumab plus Nab-paclitaxel (PTX) treatment for PD-L1-positive and locally unresectable advanced or metastatic TNBC (mTNBC) in 2019.7
The immune system plays a dual role in tumor suppression and tumor promotion during cancer development. These dual roles are collectively known as “cancer immunoediting” and include three sequential stages: elimination, equilibrium, and escape.8 During the elimination phase, the innate and adaptive immune systems work together to detect and destroy a developing tumor; however, tumor cell variants can survive the elimination phase and enter the equilibrium phase. In the equilibrium state, the adaptive immune system shapes the immunogenic environment such that tumor cells can maintain a functional dormant state, with the growth of occult tumors controlled by immune specificity. However, variant tumor cells can still emerge despite the constant pressure of immune selection. These variant tumor cells enter the escape phase, during which their growth is no longer blocked by immunity, leading to clinically significant disease.9
TNBC immunoediting is manifested by infiltration of TILs and the presence of proinflammatory cytokines, such as interferon (IFN)-γ and tumor necrosis factor (TNF)-α, which are involved in controlling tumor growth.10 Usually, breast tumor progression is accompanied by extensive stromal modifications. The mammary epithelial cells undergo genetic alterations characterized by abnormal proliferation and progress from atypical hyperplasia to ductal carcinoma in situ (DCIS). In DCIS, leukocytes in the periductal stroma are recruited and activated to interact with cancer cells so that the tumor remains the equilibrium state.11 Immune escape of TNBC can occur through various mechanisms, such as reduced immune-recognition ability, increased resistance to immune cell attack, or formation of an immunosuppressive TME.12 Immune escape is a critical step in the progression of TNBC, resulting in the development of DCIS to invasive carcinoma, and ultimately distant metastasis.
In recent research, immune-based classification of tumors has been proposed, introducing the concept of “cold” and “hot” tumors. “Cold” tumors are immunosuppressive tumors characterized by a lack of T cell infiltration, a low tumor mutation load, poor antigen presentation, and intrinsic insensitivity to T cell killing. “Hot” tumors are immunogenic tumors with high T cell infiltration and checkpoint expression. TIL levels are used to predict the responses of TNBC to chemotherapy, with increased TIL levels in the TME associated with higher rates of complete pathological remission and survival benefits for patients.10,13,14 In addition, patients with “hot” tumors benefit more from immunotherapy compared to patients with “cold” tumors.15
Increasing evidence suggests that chemotherapy combined with immunotherapy can restore immune surveillance functions.16–18 Traditional chemotherapeutic agents can create an immunogenic TME through various mechanisms, such as increasing the mutational load, upregulating the tumor antigen load, enhancing major histocompatibility class I (MHC-I) expression to promote antigen presentation, enhancing T cell recruitment to tumors or reducing immunosuppressive cells, and transforming “cold” tumors lacking immune component infiltration into “hot” tumors that are highly infiltrated by TILs and contain high cytokine levels.18,19 Subsequently, simultaneous or sequential administration of immunotherapeutic agents can enhance the T cell immune response and antitumor activity to generate an effective antitumor immune response. Therefore, chemoimmunotherapy emerges as a potential treatment option for TNBC.
The emergence of nanotechnology has provided precise and targeted treatment modalities for TNBC chemoimmunotherapy. Nanodelivery systems can prolong drug circulation times, increase drug accumulation, achieve targeted delivery and controlled release, significantly enhance antitumor immune responses, and remodel the immunosuppressive microenvironment. In this review, we discuss the immunomodulatory effects of chemotherapy and the mechanisms of chemotherapy combined with immunotherapy. Further, we introduce the commonly used nanocarriers including liposomes, polymeric nanoparticles, dendrimers, hydrogels, metal and inorganic nanoparticles, and cell-derived nanoparticles. We also summarize the nanotechnology-mediated co-delivery systems and their different drug loading modes, targets, and mechanisms. Finally, we provide some insights into the application prospects of nanoparticle-mediated chemoimmunotherapy against TNBC.
Immunomodulatory Effects of Chemotherapy
The TME is a complex system composed of tumor cells, immune cells, tumor-associated fibroblasts, extracellular matrix, cytokines, chemokines, microvasculature, lymphatic vessels, and other components.20 Thus, many immunogenic and suppressive components are present in the TME. Traditional chemotherapeutic drugs not only induce non-immunogenic apoptosis and cytotoxic killing, however, also kill tumor cells and generate cross-presenting tumor antigens that stimulate the tumor-specific immune responses by increasing tumor immunogenicity or eliminating the immunosuppressive TME.21,22 The immunomodulatory effects of chemotherapy may further enhance its antitumor effect in a synergistic manner with immunotherapy.
Chemotherapeutic Drugs Promote Tumor-Specific Immune Responses
The ability to elicit a tumor-specific immune response depends on the antigenicity and adjuvancy of cells; two essential requirements must be met simultaneously: tumor cells must (1) express antigens that can be recognized by the initial T cells and (2) deliver adjuvant-like danger signals to antigen-presenting cells (APCs) in the form of endogenous damage-associated molecular patterns.23–25 Many conventional chemotherapeutic agents enhance the tumor antigenicity and adjuvant properties of cancer cells by inducing immunogenic cell death (ICD).
Tumor-rejection antigens, including tumor-associated antigens, viral antigens, and tumor-specific antigens, are targets of antitumor T cells.12 Chemotherapeutic agents can enhance tumor antigen presentation by upregulating the expression of tumor antigens and MHC-I molecules or by increasing the recruitment of APCs. In addition, MHC-I upregulation driven by increased IFN-γ signaling, transcriptional interference, and genetic defects can enhance cancer cell antigenicity.26,27 MHC-I bound to antigenic peptides on the cancer cell surface is recognized by CD8+ T cells, which triggers tumor-specific immune responses.
ICD is a specific form of apoptosis in which an immune response is triggered against dead cell-associated antigens in an immunologically active host without an adjuvant. During the adaptive immune response and plasma membrane permeation triggered by ICD, dying tumor cells release danger signals, such as calreticulin, adenosine triphosphate, high-mobility group protein-1 (HMGB1), and heat shock proteins.28–30 The released danger signals bind to pattern-recognition receptors on APCs, such as dendritic cells (DCs), and activate DCs to phagocytose tumor-associated antigens for presentation to T lymphocytes, thereby triggering tumor-specific immune responses.31,32 These dying tumor cells are effective as endogenous vaccines. Not all chemotherapeutic agents can trigger ICD; for example, cisplatin must be combined with endoplasmic reticulum stress inducers to trigger ICD.33 Chemotherapeutic agents approved by the FDA for treating breast cancer that can trigger ICD include doxorubicin (DOX), epirubicin, mitoxantrone (MTO), and cyclophosphamide. In addition, several chemotherapeutic agents are potential ICD inducers. For example, in vitro data showed that early cell-surface exposure to calreticulin, docetaxel, PTX, carboplatin, camptothecin, and 5-fluorouracil can induce ICD.34,35
Chemotherapeutic Drugs Eliminate the Immunosuppressed TME
Chemotherapeutic drugs can interact with immune cells and exert immunomodulatory effects by promoting the activation of immune effector cells or by impeding the functions of immunosuppressive cells. Cytotoxic drugs, such as topoisomerase inhibitors and anti-microtubule agents, can directly promote DC activity and induce ICD, which indirectly promotes DC recruitment and maturation.36–39 In addition, low doses of anthracycline, 5-fluorouracil, and PTX can directly stimulate T cell activity or render tumor cells more sensitive to cytotoxic T lymphocytes (CTLs) and NK cells through mechanisms dependent on perforin and granzyme B.
Multiple suppressive components are present in the TME, including regulatory T cells (Tregs), myeloid-derived suppressor cells (MDSCs), and M2-like tumor-associated macrophages (TAMs), as well as immunosuppressive molecules, such as PD-L1 and immunosuppressive cytokines (interleukin [IL]-10 and transforming growth factor [TGF]-β), which are closely associated with tumor immune escape.12 Several studies have demonstrated that high infiltration of Tregs, MDSCs, and TAMs is associated with low survival rates of patients with TNBC.40 Chemotherapeutic drugs can modulate the activity of the anti-tumor immune response by depleting immunosuppressive cells. For example, in animal models and patients with advanced breast cancer, metronomic and a low dose of cyclophosphamide selectively reduces Tregs and induces a stable tumor-specific T cell response that promotes antitumor immunity.41,42 Additionally, Tregs express high levels of immune-checkpoint molecules, such as CTL-associated antigen 4 (CTLA-4) and chemokine receptors, which are targets of some monoclonal antibodies or small-molecule immune agents. DOX, gemcitabine, 5-fluorouracil, and PTX deplete circulating MDSCs in mouse models of breast cancer and enhance T cell functions and immune signal transduction.43,44 Macrophages can be polarized into a pro-inflammatory phenotype (M1) with a high antigen-presenting capacity and an anti-inflammatory phenotype (M2) that promotes tumor invasion and immunosuppression. Low doses of DOX and cyclophosphamide promote the polarization of M2 macrophages toward the M1 phenotype and enhance the immune response by enhancing IL-6 and IL-12 production and inducing DC, NK, and CTL activation.45
Synergistic Mechanism of Chemotherapy and Immunotherapy
The cancer-mediated immune response is a cyclical process regulated by immunostimulatory or inhibitory signals; this process is known as the cancer immune cycle. The main steps of this cycle include tumor-associated antigen release, antigen presentation by APCs, T cell activation and proliferation, T cell trafficking and tumor infiltration, T cell recognition, and tumor cell killing.46 Immunotherapy improves the antitumor immune response by activating or suppressing components of the immune system, which ultimately establishes a durable, highly active T cell response against the tumor and helps form a stable immune memory. However, the clinical application of immunotherapy is constrained by the immunosuppressive TME. For instance, cancer cells can express suppressive molecules, leading to the evasion of immune system surveillance, reduced efficacy of immunotherapeutic approaches, and ineffective monoimmunotherapy against tumor cells.47,48
Breast cancer is less immunogenic than other malignancies, and chemotherapy can reduce tumor loads and create an “immunogenic” TME. Preclinical findings suggested that chemotherapeutic and immunotherapeutic agents can function synergistically through different pathways to stimulate or suppress immune responses of different targets during the cancer immune cycle (Figure 1). Combination therapy can enhance the antitumor effect and promote rebuilding of the autoimmune system with low toxicity and durable effects. Several cancer-immunotherapy approaches have been developed, including immune-checkpoint blockade, cancer vaccines, adoptive cell therapies, and cytokine therapies.49 The following section describes combined therapeutic approaches, as well as compares and contrasts the mechanisms of action of chemotherapeutic agents with those of several common immunotherapeutic agents.
Chemotherapy Combined with Immune Checkpoint Inhibitors
Numerous inhibitory pathways present in the immune system contain immune checkpoints. For example, the immunomodulatory molecules PD-1 and CTLA-4 (expressed on activated T cells) bind to their cognate ligands to inhibit T cell functions, including proliferation, cytokine release, and cytotoxic granule secretion, thereby preventing excessive immune responses.50 Immune checkpoints can be blocked by antibodies or regulated by recombinant ligands or receptors. ICIs restore T cell function and the ability of these cells to clear cancer by inhibiting receptor-ligand interactions and eliminating inhibitory pathways that block T cell responses.51 ICIs do not directly target tumor cells but rather target lymphocyte receptors or their ligands to enhance endogenous antitumor activity. Immune checkpoint therapies have fewer toxic side effects and longer duration compared to those of chemotherapeutic agents, and have been successfully used alone and in combination to treat malignancies, such as lung cancer and melanoma. Chemotherapy leads to tumor cell death and antigen release, which initiate T cell activation, resulting in increased TIL infiltration and PD-L1 expression, development of an immunogenic TME, and an elevated antitumor effect of ICI.
The PD-1/PD-L1 signaling pathway inhibits the killing of cancer cells by T cells; when PD-1 on T cells binds to PD-L1 on the tumor cell surface, T cell activity is inhibited, T cells around the tumor undergo stalled growth or apoptosis, and immune escape occurs.51 Several clinical trials have been conducted using PD-1 and PD-L1 inhibitors to treat TNBC. The results of the KEYNOTE-086 and KEYNOTE-012 trials showed that treatment with the anti-PD-L1 (aPD-L1) agent pembrolizumab alone for mTNBC showed durable antitumor activity and a manageable safety profile with objective response rates of 5–22% but did not improve the overall survival of patients with mTNBC.52–54 The results of several preclinical and clinical studies showed that chemotherapy reduces tumor resistance to immune checkpoint blockers, and the results of the TONIC trial confirmed that administering the anti-PD-1 agent nivolumab after chemotherapy induction significantly increased the objective response rate.55 The subsequent Phase III trial IMpassion130 demonstrated that atezolizumab combined with Nab-PTX prolonged progression-free survival and improved overall survival rates in patients with metastatic TNBC, with a more significant clinical benefit observed in the PD-L1-positive subgroup.5 In the phase III KEYNOTE-355 trial, the addition of pembrolizumab to chemotherapy for mTNBC showed similar progression-free survival effects.56 In 2019, the FDA approved atezolizumab in combination with albumin PTX as a first-line treatment for PD-L1-positive, inoperable, metastatic TNBC.
Anti-CTLA-4 antibodies inhibit T cell initiation and activation, induce T cell unresponsiveness, and negatively regulate the immune response. In addition, several potential immune checkpoint molecules, such as T cell immunoglobulins and mucin-containing structural domain-3, indoleamine-pyrrole 2,3-dioxygenase (IDO), and lymphocyte activation gene 3, along with their associated antibodies, have been evaluated in several preclinical experiments. A previous study showed that IDO was highly expressed in TNBC and associated with a poor prognosis.57 In a mouse model of breast cancer, IDO inhibitors improved the response to chemotherapy and exerted synergistic effects with multiple chemotherapeutic agents to cause the regression of tumors that were ineffectively treated with monotherapy.58 Furthermore, most PD-L1-positive tumors co-express IDO, and interference with the IDO pathway by the immune checkpoint inhibitor aPD-1 is not redundant; thus, IDO inhibitors can synergistically enhance the immune response to PD-1 inhibitors.59
Chemotherapy Combined with Cytokines
Cytokines are small-molecule proteins released by cells or bound to membranes and play important roles in regulating the growth, differentiation, and activation of immune cells. Cytokines can directly induce apoptosis or promote immune responses by host cells to exert anti-tumor effects. They can also mediate immune escape and promote tumor growth by recruiting suppressive immune cells. Commonly used cytokines include TNFs, ILs, IFNs, and granulocyte-macrophage colony-stimulating factors. However, cytokines are rapidly cleared in vivo; thus, achieving good antitumor effects with cytokines alone is difficult. Moreover, high doses of cytokines cause serious systemic toxicity.
The antitumor effects of cytokines can be enhanced when they are used in combination with chemotherapeutic agents, and cytokine-based immunotherapy can improve the sensitivity of tumor cells to chemotherapy and reverse tumor drug resistance. For example, most breast cell lines are insensitive to tumor necrosis factor-related apoptosis-inducing ligand (TRAIL)-induced apoptosis; however, treatment with TRAIL in combination with DOX or 5-fluorouracil can synergistically activate caspase-induced apoptosis.60 IL-2 promotes the expansion and activation of NK and T cells, and the combination of IL-2 and cyclophosphamide can reduce the tumor load, enhance the tumor sensitivity to cellular immunolysis, and/or reduce chemotherapy-induced suppressor cell activity.42 However, the TNBC microenvironment is enriched in inhibitory cytokines, such as IL-10 and TGF-β. TGF-β inhibitors, can block PTX-induced cancer stem-like cell expansion by promoting IL-8 release and preventing TNBC recurrence.61 Chemokine CXCR antagonists can eliminate intra-tumor fibrosis by increasing T cell infiltration; reducing the levels of MDSCs, Tregs, and pro-metastatic cytokines; and synergizing with chemotherapy-induced ICD to increase CD8+ T cell recruitment, thereby further enhancing anti-tumor immunity.62
Chemotherapy Combined with Immune Adjuvants
Adjuvants are molecules that promote specific immune responses by activating pathogen pattern-recognition receptors including Toll-like receptors (TLRs), nucleotide oligomerization domain-like receptors, retinoic acid-inducible gene I-like receptors, C-type lectin receptors, and a series of intracellular DNA sensors. Combination treatment with chemotherapy and immune adjuvants synergistically enhances DC activation, increases helper and cytotoxic T cell levels, and enhances antigen-specific T cell responses.
TLRs comprise a family of pattern recognition receptors important for natural immunity and bind ligands with a high degree of specificity. TLR signaling can control immune cell activation, immune cell maturation, and cytokine secretion as well as influence tumor proliferation and metastasis. Phase I and II clinical trials have been conducted using immune adjuvants alone or as therapeutic vaccine adjuvants to enhance antitumor immune responses. Monophosphoryl lipid A, a lipopolysaccharide derivative, is a TLR4 agonist that stimulates immune response through myeloid differentiation factor 88 (MyD88) or Toll/IL-1 receptor containing adaptor molecule (TRIF) pathway.63 MyD88 pathway activates signal transduction factors and finally leads to the activation of nuclear factor-kappa B (NF-κB) and the production of pro-inflammatory factors. TRIF induces the expression of IFN-β through a MyD88-independent pathway, causing associated inflammatory responses. Unmethylated cytosine-phosphoguanine (CpG) motifs are TLR9 agonists that induce a series of innate and acquired immune responses by turning on the NF-κB and mitogen-activated protein kinase signaling pathways, directly inducing DC maturation and activation, and producing pro-inflammatory cytokines and chemokines that indirectly activate NK cells and T cells.64 Immune adjuvants such as the TLR3 ligand polyinosinic: polycytidylic acid, TLR7 agonist imiquimod R-837, and TLR7/8 agonist resiquimod R848 can promote DC activation and produce Th1 cells and CTLs, which enhances anticancer activity.64–66
Stimulator of interferon genes (STING) is an endoplasmic reticulum-sequestration protein that serves as an important signaling molecule in intrinsic immunity. Accumulation of cytoplasmic DNA in response to DNA viruses, bacterial pathogens, and chemotherapy activates cyclic GMP-AMP synthase to produce cyclic GMP-AMP and cyclic dinucleotide, bind and activate STING, and produce type I IFNs and various other cytokines, such as IL-6, IL-6, and IFN regulatory factor 3 via nuclear factor-κB and initiation of the IFN regulatory factor 3 pathway.67 STING can initiate intrinsic immunity by recognizing exogenous DNA or releasing DNA from itself through the cyclic GMP-AMP synthase pathway or by promoting DC maturation for antigen presentation and T cell activation. In combination with chemotherapy or immunotherapy, STING agonists can activate STING in tumor cells or tumor-infiltrating immune cells to produce an immunostimulatory effect.
Nanoparticle-Mediated Chemoimmunotherapy
The main challenge in chemoimmunotherapy is targeting both cancer and immune cells simultaneously and specifically. Application of nanoparticle-mediated drug-delivery systems loaded with chemotherapeutic drugs and immunotherapeutic agents can overcome the poor solubility and low bioavailability of free drugs, prolong the drug circulation time, increase drug accumulation, and deliver drugs to specific targets by responding to specific pH and temperature conditions in tumor tissues or the TME.68,69 Commonly used nanocarriers include liposomes, polymeric nanoparticles, dendrimers, hydrogels, metal and inorganic nanoparticles, and cell-derived nanoparticles.
In addition, chemoimmunotherapy mediated using nano-delivery systems can achieve synergistic drug delivery through different drug-delivery modes. “Free drug+nano” and “nano+nano” delivery modalities enable adjustments to prescriptions and dosing intervals but do not effectively control the drug distribution and onset of action in the body and may lead to systemic toxicity. Currently, chemotherapeutic drugs loaded onto a nanoplatform and immunotherapeutic agents administered in the free form are commonly used in nanomedicine-mediated chemoimmunotherapy. This method has good prospects for clinical applications, as it reduces the side effects of combination therapy and the agents are easy to prepare. The co-encapsulation method can control drug accumulation in a tumor in an appropriate ratio and can control drug release to specific locations in response to tumor-specific conditions, such as pH or temperature, to enable the spatiotemporal control of drug delivery, although the preparation process is complex. Table 1 summarizes the representative applications and mechanisms of nanoparticle-mediated co-encapsulation methods for immunochemotherapy in TNBC.
![]() |
Table 1 Applications and Mechanisms of Nanoparticle-Mediated Co-Encapsulation Methods for Chemoimmunotherapy in TNBC |
Liposomal Nanoparticles
Liposomes are nano- or micro-sized phospholipid vesicles consisting of a hydrophilic core and lipid bilayer. Liposomes are ideal carriers for co-delivering small-molecule drugs and some biomacromolecules. Liposomes are highly biocompatible, can prolong the half-life of drugs, and improve the encapsulation efficiency and stability of drug-delivery systems. Typically, hydrophilic small-molecule drugs are encapsulated in an inner core, whereas hydrophobic therapeutics are encapsulated in a hydrophobic phospholipid bilayer or adsorbed to the lipid surfaces through physical or chemical interactions. Therefore, liposomes are effective delivery vehicles for oligonucleotides, peptides, and small-interfering RNA-based gene therapies. In addition, some cationic liposomes are immunostimulatory adjuvants that stimulate specific immune responses.94
Liposomes also enable the co-delivery of chemotherapeutic drugs and immunotherapeutic agents to achieve more effective synergistic immunomodulatory effects. Lu et al, designed a codelivery system by conjugating the IDO inhibitor indoximod (IND) to a single-chain phospholipid, followed by mixing with cholesterol and 1.2-distearoyl-sn-glycero-3-phosphoethanolamine-N-[methoxy(polyethylene glycol)-2000] to construct liposomes, which were remotely loaded with DOX via a proton gradient.59 DOX/IND-liposomes improved the pharmacokinetics and tumor uptake of both drugs and significantly enhanced the antitumor immune response by simultaneously inducing ICD and inhibiting the IDO pathway in a 4T1 tumor model. Combination treatment with aPD-1 further enhanced the antitumor immune response and eradicated lung metastasis. Similarly, Mei et al, constructed MTO/IND liposomes using the conventional lipid film-hydration method.71 MTO has a stronger ICD-inducing effect compared to DOX, is used less frequently, and provides another therapeutic option for DOX-resistant patients. MTO/IND liposomes inhibited tumor growth in mouse models of colon, breast, and renal cancer.
Modified liposomes can target specific sites and exert therapeutic effects through different pathways. For example, Chen et al designed matrix metalloprotease 2 responsive nanoparticles to deliver PTX and anti-CD47 by detachable immune liposomes, which target to tumor cells and M2-like TAMs respectively73 (Figure 2). For example, Deng et al developed a folic acid (FA)-modified pH-responsive liposome (PEG-FA-liposome) loaded with DOX, which targeted 4T1 breast cancer cells and M2-TAMs through FA receptor-mediated endocytosis.95 In addition, liposomal-delivery systems can precisely deliver chemotherapeutic and immunotherapeutic agents to their target cells. For example, Du et al, designed nanosatellites ([email protected]@Res) that disintegrated in an acidic tumor environment into resveratrol-loaded zwitterionic liposomes ([email protected]); these agents reversed the phenotype of M2-TAMs and DOX-loaded HFn nanoparticles ([email protected]), and thus targeted tumor cells to exert anti-tumor effects.70 Jiang et al, designed Gelipo with a liposomal core and crosslinked-gel shell to deliver TRAIL and DOX to extracellular and intracellular targets, respectively.72 TRAIL in the hyaluronan (HA) shell was released in the extracellular HA-enzyme-enriched microenvironment and exogenously induced apoptosis by binding to membrane receptors to activate the caspase 3-signaling pathway. Thus, peptide-modified liposomes can deliver DOX to the nucleus to activate intrinsic apoptotic pathways.
![]() |
Figure 2 (A) Tumor-associated macrophages (TAMs) promoting tumor growth in TNBC and (B) PTX-ILips designed for the enhanced efficacy of immunochemotherapy against TNBC. Notes: Reprinted with permission from Chen M, Miao Y, Qian K et al. Detachable Liposomes Combined Immunochemotherapy for Enhanced Triple-Negative Breast Cancer Treatment through Reprogramming of Tumor-Associated Macrophages. Nano Letters. 2021;21(14):6031–6041. Copyright 2021, American Chemical Society.73 |
Polymer Micelles
Polymer micelles are delivery systems consisting of amphiphilic block copolymers with a hydrophilic shell surrounding a hydrophobic core structure, with average diameters of 5–100 nm. At concentrations below the critical micelle concentration, the micelles can break down into free monomers. Polymeric micelles have a low critical micelle concentration, which increases their circulation time. Hydrophobic small-molecule drugs can be encapsulated in the hydrophobic core of micelles, and hydrophilic drugs or proteins can be encapsulated in nanoparticles or adsorbed on their surfaces through physical or chemical means, such as electrostatic, hydrogen bonding, and hydrophobic interactions.
Polymer micelles confer specific functions to micelles by linking block copolymers with specific functions. Liu et al, designed polymeric micelles that self-assembled from hypoxia-sensitive block copolymers incorporated both DOX and ICG.96 The block copolymer consisted of 6-(2-nitroimidazolyl) hexylamine moieties that targeted anoxic tumor environments. Transforming the micelles from a hydrophobic to a hydrophilic state via reduction under hypoxic conditions activated DOX- and ICG-mediated chemotherapy and photodynamic therapy/photothermal therapy, which together induced ICD in tumor cells. In addition, combination treatment with CpG and aCTLA4 amplified the immune response to effectively eliminate the primary tumor and distant lung metastases. Lan et al, developed a novel bifunctional immunostimulatory polymer (DOX/PEG2k-Fmoc-1-methyl tryptophan micelles) to simultaneously deliver 1-methyl tryptophan and DOX.75 Micelle-based drug-delivery systems can increase drug accumulation in tumors and prolong circulation times, significantly enhancing the antitumor immune response.
Polymer Nanoparticles
Polymer nanoparticles are nano-delivery systems in which a drug is physically dispersed, dissolved, or chemically bound to the polymer chains and can be divided into nanospheres or nanocapsules according to the preparation method.97 Nanospheres are solid colloidal particles with a drug dispersed in a polymer matrix and a diameter of 100–200 nm. Nanocapsules are vesicle systems formed by a monolayer of polymeric film surrounding a hydrophilic or lipophilic drug-containing core and are typically 100–300 nm in diameter. Polymer nanoparticles offer the advantages of targeting, controlled drug release, improved solubility of insoluble drugs, improved efficacy, and reduced toxicity, thereby enabling the delivery of combinations of drugs with different physicochemical properties.
Liang et al, used dialysis to prepare spherical nanoparticles ([email protected]) formed by self-assembly of triblock copolymers, which enabled the delivery of the chemotherapeutic drug SN38 and STING agonist DMXAA.86 SN38 acts as a hydrophobic core in PS3D1 nanoparticles, assembled through covalent bonds, and the carboxyl group in DMXAA interacts electrostatically with 2-(diethylamino)ethyl methacrylate for loading into the hydrophobic core. The nanoparticles induced ICD and enhanced the secretion of the chemokine CCL4. DMXAA also induced conversion of the immunosuppressive TME to an immunogenic TME by activating the STING pathway of DCs, leading to the production of type I IFNs, which further enhanced the proliferation and infiltration of antigen-specific CD8+ T cells. [email protected] showed potent therapeutic effects in mouse models of breast, melanoma, and colon cancers and further enhanced the antitumor response in combination with anti-PD-1 treatment.
Polymers commonly used to construct nanoparticles include polyester homopolymers; poly(lactic-co-glycolic acid) and polylactic acid are FDA-approved polymeric materials that are biodegradable and biocompatible. Many polymer nanoparticles have been designed to undergo structural changes (size changes, charge conversions, or shell-core separation) in response to stimuli in the TME, thus enabling targeted and programmed delivery of different drugs. pH-, temperature-, enzyme-, and light-sensitive block copolymers dissociate under specific conditions, allowing for controlled drug release and providing ideas for intelligent drug delivery. For example, Hu et al, developed nanoparticles for co-delivering PTX and IL-12 with an acid-sensitive material polylactic acid copolymer (mPEG-Dlinkm-PDLLA) as the core and Pluronic F127 with a low-temperature expansion effect as a hydrophilic sponge shell.84 In the acidic TME, the pH-sensitive bridge chain (Dlinkm) is disrupted, which promotes the release of PTX and IL-12. Zhu et al, prepared nanoparticles (CaCO3@poly(lactic-co-glycolic acid)-PEG) via a CaCO3-assisted double-emulsification method and simultaneously encapsulated DOX and the IDO inhibitor aNLG919 into the cavity and polymeric layer, respectively.79 CaCO3 decomposed and neutralized the acidic TME. The released DOX and aNLG919 caused ICD and inhibited the IDO1 pathways, respectively, which synergistically promoted host-specific antitumor immunity by increasing CTL infiltration and eliminating Tregs.
In addition, multifunctional polymer carriers can be obtained by modifying the surface of polymers. Specific targeting molecules (such as folic acid, transferrin, and peptides) are coupled to nanoparticle surfaces to bind to specific receptors on the cell surface, enabling the nanoparticles to enter cells via endocytosis to achieve drug accumulation and targeted delivery. For example, HA shows good biocompatibility and can bind to the CD44 receptor on the surface of breast cancer cells and enter the cells through endocytosis to achieve targeted drug delivery. Wang et al developed a detachable core–shell nanoplatform ([email protected]/CXB) by coupling a hydrophilic [email protected] prodrug with a hydrophobic 3-diethylaminopropyl isothiocyanate (DEAP) via an MMP2-substrate peptide and loading CXB through a self-assembly method.81 [email protected] triggered ICD by precisely targeting CD44, which increased tumor immunogenicity. CXB increased the recruitment of DCs and T cells to the TME by inhibiting prostaglandin E2 production. In addition, CXB eliminated the physical barrier between CTLs and tumor cells by reducing CXCL12 production, resulting in a potent anti-tumor effect (Figure 3). Similarly, several researchers have designed HA shell-based nanoparticles80,83 that decompose in response to the acidic microenvironment and programmatically release chemotherapeutic drugs and immunotherapeutic agents to exert combined chemoimmunotherapeutic effects, significantly enhancing the anti-tumor activity.
![]() |
Figure 3 (A) Synthesis of the detachable vesicles. (B) Schematic depiction of the antitumor immunotherapy elicited by vesicles in vivo. Notes: Reprinted with permission from Wang L, Ding K, Zheng C et al. Detachable Nanoparticle-Enhanced Chemoimmunotherapy Based on Precise Killing of Tumor Seeds and Normalizing the Growing Soil Strategy. Nano Lett. 2020;20(9):6272–6280. Copyright 2020, American Chemical Society.81 |
Dendrimers
Dendrimers are hyperbranched spherical polymers formed by hydrophobic cores, repeating monomers, and functional peripheral groups. They are frequently used as materials for drug loading because of their large internal cavities and controlled physicochemical properties. Dendrimers are degraded by glycosylation, acetylation, PEGylation, or peptide modification. Dendrimers, such as polypropyleneimine (PEI) and polyamidoamine dendrimers are widely used. Shen et al, constructed pH-sensitive nanocarriers (BLZ-945SCNs/Pt) to deliver platinum prodrugs and BLZ-945 (a colony-stimulating factor 1 receptor inhibitor) to tumor cells and TAMs, respectively, which were self-assembled from platinum-prodrug-conjugated polyamidoamine dendrimers, whereas BLZ-945 was encapsulated in the hydrophobic domain.85 Delivery systems can lead to TAM depletion and increased tumor immunogenicity, which inhibit tumor growth and suppresses metastasis (Figure 4).
![]() |
Figure 4 Mechanism of spatial delivery of BLZ-945 and Pt-prodrug to TAMs and tumor cells. Notes: Reprinted with permission from Shen S, Li HJ, Chen KG et al. Spatial Targeting of Tumor-Associated Macrophages and Tumor Cells with a pH-Sensitive Cluster Nanocarrier for Cancer Chemoimmunotherapy. Nano Lett. 2017;17(6):3822–3829. Copyright 2017 American Chemical Society.85 |
Hydrogels
Hydrogels are three-dimensional polymer networks prepared by cross-linking hydrophilic polymer chains in an aqueous medium. Hydrogels are highly biocompatible, biodegradable, redox-responsive, and pH-responsive, making them ideal carriers for small-molecule drugs and biomolecules.98–100 Materials used for hydrogel formation include synthetic polymers or purely natural polymers such as chitosan, gelatin, and hyaluronic acid. In addition, hydrogels can deliver antigens as cancer vaccines to activate DCs or macrophage-mediated active immunity, and can be used as vehicles to continuously deliver T cells for passive tumor immunotherapy.101
Wang et al, developed reactive oxygen species (ROS)-responsive scaffolds that not only allow the local release of gemcitabine and aPD-L1 with distinct release kinetics but also act as ROS scavengers within the TME to deplete ROS and block M2 macrophage differentiation to improve therapeutic efficacy.87 ROS-responsive scaffolds enhanced tumor immunogenicity, increase TIL counts and PD-L1 expression, and decrease MDSC and TAM levels. aPD-L1 facilitated subsequent therapeutic immune responses. In addition, ROS-responsive scaffolds synergistically promoted immune-mediated tumor regression and prevented tumor recurrence after initial resection in mouse models with B16F10 melanoma and 4T1 mammary tumors.
Metal Nanoparticles
The advantages of metal nanomaterials include their good histocompatibility, stability, and photothermal effects. Because of the magnetic, optical, thermal, and electrical properties of metal nanoparticles, they can directly kill tumor cells and induce ICD through photothermal effects or other methods. Nanomaterials, such as gold, silver, copper, iron, gadolinium, and ruthenium show high potential for tumor imaging, drug delivery, and gene therapy. In addition, metal nanoparticles loaded with chemotherapeutic drugs can interfere with cell metabolism, inhibit cell-proliferation signals, and induce apoptosis, providing a foundation for precision treatment of TNBC.
Metal nanoparticles have high photothermal efficiency and good biocompatibility, and are commonly used as carriers for photothermal, photodynamic, and magnetothermal therapies. To induce ICD, Dong et al, developed nanocomposites (FA-CUS/[email protected]) with mesoporous CuS nanoparticles as drug carriers and photosensitizers loaded with low doses of DTX.90 The nanoparticle surface was modified with the tumor-targeting ligand FA and polyethylenimine–protoporphyrin IX (PEI-PpIX) conjugates. Further, CpG was anchored to the nanoparticles to develop a new integrated synergistic treatment strategy involving photothermal therapy, photodynamic therapy, chemotherapy, and immunotherapy. DTX induced ICD and promoted MDSC polarization. CpG specifically bind with TLR9 in DCs and macrophages, which induced Th1 cell activation and pro-inflammatory cytokine production, as well as increased CTL infiltration and IFN-γ secretion, resulting in tumor cell death. Combination with aPD-L1 further enhanced the immunotherapeutic effect and enabled systemic treatment.
Metal nanoparticles possess fluorescence properties that enable in vivo fluorescence imaging, which can be used to track drug release in real-time. Wang used MnO2-CpG-silver nanocluster-DOX (MCAD) as conjugates to enhance immunochemotherapy against tumors. Nanocarriers that couple DOX to MnO2 nanosheets via π–π interactions can significantly enhance CpG-mediated tumor immune responses by inducing ICD and eliminating the immunosuppressive microenvironment.88 Furthermore, based on the fluorescence properties of CpG-silver nanoclusters and T1 magnetic response imaging (MRI) properties of Mn2+, MCAD enabled MRI and in vivo fluorescence imaging of tumors, which allowed for real-time tracking of CpG release and comprehensive assessments of treatment efficacy. Zhou et al, designed Mn3O4@Au-dsDNA/DOX nanoparticles to co-deliver chemotherapeutic agents and STING activators. The Mn3O4 nanoparticles modified the hypoxic TME by generating O2, whereas the released Mn2+ was used for MRI imaging to assess nanoparticle accumulation in tumors.89
In a recent study, Yang et al designed nanoparticles Pt(IV)/CQ/PFH NPs-DPPA-1 that improved the effect of chemoimmunotherapy combined with platinum(IV) and anti-PD-L1 peptide (Figure 5). The nanoparticles released platinum(IV) and chloroquine (CQ), which inhibited protective autophagy while increasing intracellular content of ROS in tumor cells, improved TME by increasing the proportion of mature DCs and M1 macrophages. In addition, perfluorooctane (PFH) can serve as an imaging agent, achieving monitoring therapy corresponding to ultrasound.102
![]() |
Figure 5 Schematic illusion of Pt(IV)/CQ/PFH NPs-DPPA-1 assisted by ultrasound for augmenting chemoimmunotherapy of breast cancer. Notes: Reprinted with permission from Yang X, Zhao M, Wu Z et al. Nano-ultrasonic contrast agent for chemoimmunotherapy of breast cancer by immune metabolism reprogramming and tumor autophagy. ACS Nano. 2022;16(2):3417–3431.102 |
Silica Nanoparticles
Mesoporous silica nanomaterials (MSNs) are characterized by their ordered macroporous structure and easy chemical modification. MSNs have good prospects for clinical applications because of their high drug loading, good biocompatibility, and controllable structures. Zheng et al, found that using MSNs as carriers, DOX-mediated antitumor immune responses could be amplified by enhancing permeability and retention effects.103 They synthesized logical DOX-releasing MSNs ([email protected]), which prolonged the drug-circulation time and induced antitumor immune responses by promoting DC maturation and antitumor cytokine release. Zhang loaded diselenide-bridged mesoporous organosilica nanoparticles with a chemotherapeutic ruthenium compound (KP1339) via adsorption, which not only controlled drug release in response to glutathione in the TME but also enhanced endoplasmic reticulum stress and amplified the ICD effect.104 These nanoparticles were successfully used to eliminate breast cancer tumors in situ and lung metastases in combination with aPD-L1. MSNs are commonly used as carriers for PTT. Cheng et al, utilized CuS nanoparticles with a high photothermal conversion efficiency deposited in situ in dendritic macroporous mesoporous silica (DLMSN) loaded with the immune adjuvant R848 and anti-PD-1 peptide AUNP-12 to treat metastatic TNBC in vitro and in vivo.105
Biomimetic Nanoparticles
Biomimetic nanoparticles are nanomaterials that mimic the composition and structural characteristics of natural biological materials. Cell-derived nanovesicles are spherical membrane vesicles that retain their biomimetic properties, show good biocompatibility, have specific cell-targeting abilities, and are natural drug delivery vehicles. Wu et al, prepared nanovesicles (NV-DOX) by co-extruding DOX and DC2.4 cells.93 NV-DOXIL-2 /IFN-γ was obtained by the adsorption of cytokines IFN-γ and IL-2 onto NV-DOX using a simple solvent-free encapsulation method with high encapsulation efficiency that did not affect cytokine bioactivity. These NVs enhanced DC maturation in tumor-derived lymph nodes (TDLNs), promoted infiltration and activation of CTLs and NK cells, and increased the recruitment of neutrophils in the TME to significantly inhibit primary tumor growth and lung metastasis in TNBC.
Hu et al, prepared biomimetic hybrid nanovesicles ([email protected]) loaded with DOX by fusing artificial liposomes with tumor-derived nanovesicles106 (Figure 6). Tumor-derived nanovesicles retain both the homologous targeting ability of cancer cells as well as tumor antigens and endogenous danger signals, which can be used as vaccines to stimulate the body to generate specific immune responses and synergize with DOX-induced ICD to exert anti-tumor effects. [email protected] showed good antitumor effects in melanoma, lung, and breast cancer in mice and enhanced antitumor effects in combination with aPD-1, leading 33% of mice to be tumor-free. Thus, biomimetic nanovesicles may be ideal natural bio-nanoplatforms with high potential for cancer therapy.
![]() |
Figure 6 (A) Preparation of [email protected] by the cofusion of TNVs with artificial liposomes. (B) Mechanism of immunochemotherapy based on the [email protected] for tumor suppression. Notes: Reprinted with permission from Hu M, Zhang J, Kong L et al. Immunogenic Hybrid Nanovesicles of Liposomes and Tumor-Derived Nanovesicles for Cancer Immunochemotherapy. ACS Nano. 2021;15(2):3123–3138. Copyright 2021 American Chemical Society.106 |
Cell membrane-camouflaged nanoparticles have tumor-targeting abilities and extended stability in the circulation. These factors preserve the biological properties of cells with immune-escape capability, improving both the drug-delivery efficiency and enhancing biosafety. For example, Du et al, constructed NK cell membrane-coated nanohybrids formed via self-assembly of PEGylated oxaliplatin-1-MT which preserved tumor tropism and the ability to induce macrophage polarization.92 The oxaliplatin in the nanoparticles triggered ICD and synergized with 1-MT to exert anti-tumor immune responses, reduce Tregs, and increase the activity of effector T cells, which transformed the tumor immune microenvironment from a cold to a hot environment. Li et al, prepared bionanoplasmic nanoplatforms for MDSC inhibition by encapsulating neutrophil membranes in polymer nanoparticles.107 Neutrophils are phenotypically and morphologically similar to polymorphonuclear MDSCs can neutralize cytokines released by tumors; and can disrupt the expansion, recruitment, and activation of polymorphonuclear MDSCs. Using nanodrugs composed of 4T1 cell membranes wrapped around berberine (a regulator of HMGB1-TLR4) and DOX self-assembly, the resulting bionanodrug homologously targeted tumor cells and inhibited tumor growth and metastasis by blocking the TLR4 pathway.
Exosomes
Exosomes are extracellularly derived phospholipid nanovesicles that mediate the delivery of bioactive molecules to specific tissues. Exosomes can be produced by all types of cells. They are 50–150 nm in diameter, have homing capabilities, and can efficiently traverse the endothelial system to accumulate at specific sites, making them effective carriers for cytotoxic drug delivery, gene therapy, and biotherapeutics.108 Exosomes improve the therapeutic efficacy of loaded drugs, such as chemotherapeutic agents and natural small-interfering RNA compounds. They can also be used as therapeutic cell-free vaccines to activate innate and adaptive immune responses and restore immune surveillance. Exosomes derived from cancer cells or immune cells are immunogenic and participate in anti-tumor immune responses. For example, exosomes secreted by M1 macrophages increase the establishment of a pro-inflammatory environment and enhance the anti-tumor effects of PTX by activating the nuclear factor-κB pathway and increasing the expression levels of caspase-3 and pro-inflammatory Th1-type cytokines.109 Exosomes, as natural carriers, avoid phagocytosis (extending their half-life in the blood) and show optimal biocompatibility, making them effective for treating cancer cells.
Conclusion
Breast cancer is less immunogenic than other types of cancer because it lacks sufficient T cell infiltration, and the efficacy of immunotherapy alone is limited. However, TNBC has a higher response rate to immunotherapy than other subtypes of breast cancer: (1) TNBC is characterized by larger numbers of TILs. (2) TNBC has higher levels of PD-L1 expression in both tumor and immune cells, providing a direct target for ICI treatment. (3) TNBC has more non-synonymous mutations that generate tumor-specific neoantigens, which activate neoantigen-specific T cells and generate antitumor immune responses. Chemoimmunotherapy combines chemotherapy and immunotherapy to synergistically elicit anti-tumor effects by inducing immunogenic cancer cell death, enhancing antigen presentation, eliminating the immunosuppressive microenvironment, and inducing the activation of immune effector cells to convert cold tumors into hot tumors.
The dose, sequence, and route of administration can strongly impact the antitumor effects of treatments. Preclinical data showed that low-dose rhythmic modes of administration are immunostimulatory, whereas high-dose chemotherapy leads to overall immune suppression. Theoretically, chemotherapeutic agents should be applied first to stimulate the body’s immune response, after which immunotherapy should be applied to enhance antitumor immunity. Therefore, nanoparticle-mediated programmed drug delivery shows better performance in combination therapy, and some preclinical trials confirmed the efficacy of programmed combination delivery of chemoimmunotherapeutic agents. However, the results of some trials suggest that ipilimumab followed by Nab-PTX plus bevacizumab is more effective than Nab-PTX combined with bevacizumab followed by ipilimumab in patients with BRAF-wild-type metastatic melanoma; therefore, the sequence of immunochemotherapy administration must be evaluated in preclinical and clinical trials.
Nanoparticle-mediated chemoimmunotherapy against breast cancer is an emerging area of research with broad clinical application prospects. Different nanomaterials have their own advantages. For instance, liposomes have better biocompatibility and lower toxicity, and some liposomal nanoparticles, such as liposomal doxorubicin, have been applied in adjuvant and neoadjuvant therapy of TNBC. However, improving the encapsulation rate of multiple drugs is a problem that needs to be solved. Polymeric nanoparticles are ideal carriers for smart drug delivery, however, they also have the problem of a more complex synthesis process. Metal nanoparticles can be used as tracers but with certain toxic side effects. Biomimetic nanoparticles have good targeting properties, and can be used as nanovaccines to activate specific immune responses, and yet their application to clinical treatment requires extensive experimental validation.
In summary, TNBC immunochemotherapy involves applying the immunostimulatory effect of chemotherapy to generate an immunogenic TME, in combination with immunotherapy to synergistically amplify the immune response through different pathways and synergistically enhance antitumor treatment effects. Nanosystem-mediated immunochemotherapy: 1) reduces the toxic side effects of combination therapy by controlling the drug dose and administration time; (2) improves therapeutic efficiency by targeting and responding to environmental stimuli with an intelligent delivery system designed to deliver both drugs to specific sites separately; and (3) involves chemotherapeutic drugs and immunotherapeutic agents that regulate the immune response through their respective pathways, significantly enhancing the therapeutic effect on primary tumors and metastatic lesions, which is promising for TNBC treatment.
Acknowledgments
This work was supported by Jilin Provincial Research Foundation of China Grants 2018SCZ014, 2019SCZ049, YDZJ202201ZYTS241, and research project of Bethune plan of Jilin University 2018B19.
Disclosure
The authors report no conflicts of interest in this work.
References
1. Dent R, Trudeau M, Pritchard KI, et al. Triple-negative breast cancer: clinical features and patterns of recurrence. Clin Cancer Res. 2007;13(15 Pt 1):4429–4434.
2. Cheang MC, Voduc D, Bajdik C, et al. Basal-like breast cancer defined by five biomarkers has superior prognostic value than triple-negative phenotype. Clin Cancer Res. 2008;14(5):1368–1376.
3. Harbeck N, Gnant M. Breast cancer. Lancet. 2017;389(10074):1134–1150.
4. Mittendorf EA, Philips AV, Meric-Bernstam F, et al. PD-L1 expression in triple-negative breast cancer. Cancer Immunol Res. 2014;2(4):361–370.
5. Schmid P, Adams S, Rugo HS, et al. Atezolizumab and nab-paclitaxel in advanced triple-negative breast cancer. N Engl J Med. 2018;379(22):2108–2121.
6. Adams S, Diamond JR, Hamilton E, et al. Atezolizumab plus nab-paclitaxel in the treatment of metastatic triple-negative breast cancer with 2-year survival follow-up: a phase 1b Clinical Trial. JAMA Oncol. 2019;5(3):334–342.
7. Narayan P, Wahby S, Gao JJ, et al. FDA approval summary: atezolizumab plus paclitaxel protein-bound for the treatment of patients with advanced or metastatic TNBC whose tumors express PD-L1. Clin Cancer Res. 2020;26(10):2284–2289.
8. Schreiber RD, Old LJ, Smyth MJ. Cancer immunoediting: integrating immunity’s roles in cancer suppression and promotion. Science. 2011;331(6024):1565–1570.
9. O’Donnell JS, Teng MWL, Smyth MJ. Cancer immunoediting and resistance to T cell-based immunotherapy. Nat Rev Clin Oncol. 2019;16(3):151–167.
10. Denkert C, von Minckwitz G, Darb-Esfahani S, et al. Tumour-infiltrating lymphocytes and prognosis in different subtypes of breast cancer: a pooled analysis of 3771 patients treated with neoadjuvant therapy. Lancet Oncol. 2018;19(1):40–50.
11. Gil Del Alcazar CR, Aleckovic M, Polyak K. Immune escape during breast tumor progression. Cancer Immunol Res. 2020;8(4):422–427.
12. Jhunjhunwala S, Hammer C, Delamarre L. Antigen presentation in cancer: insights into tumour immunogenicity and immune evasion. Nat Rev Cancer. 2021;21(5):298–312.
13. West NR, Milne K, Truong PT, Macpherson N, Nelson BH, Watson PH. Tumor-infiltrating lymphocytes predict response to anthracycline-based chemotherapy in estrogen receptor-negative breast cancer. Breast Cancer Res. 2011;13(6):R126.
14. Loi S, Drubay D, Adams S, et al. Tumor-infiltrating lymphocytes and prognosis: a pooled individual patient analysis of early-stage triple-negative breast cancers. J Clin Oncol. 2019;37(7):559–569.
15. Galon J, Bruni D. Approaches to treat immune hot, altered and cold tumours with combination immunotherapies. Nat Rev Drug Discov. 2019;18(3):197–218.
16. Smyth MJ, Ngiow SF, Ribas A, Teng MW. Combination cancer immunotherapies tailored to the tumour microenvironment. Nat Rev Clin Oncol. 2016;13(3):143–158.
17. Apetoh L, Ladoire S, Coukos G, Ghiringhelli F. Combining immunotherapy and anticancer agents: the right path to achieve cancer cure? Ann Oncol. 2015;26(9):1813–1823.
18. Bracci L, Schiavoni G, Sistigu A, Belardelli F. Immune-based mechanisms of cytotoxic chemotherapy: implications for the design of novel and rationale-based combined treatments against cancer. Cell Death Differ. 2014;21(1):15–25.
19. Chen G, Emens LA. Chemoimmunotherapy: reengineering tumor immunity. Cancer Immunol Immunother. 2013;62(2):203–216.
20. Mittal S, Brown NJ, Holen I. The breast tumor microenvironment: role in cancer development, progression and response to therapy. Expert Rev Mol Diagn. 2018;18(3):227–243.
21. Salas-Benito D, Perez-Gracia JL, Ponz-Sarvise M, et al. Paradigms on immunotherapy combinations with chemotherapy. Cancer Discov. 2021;11(6):1353–1367.
22. Luo Q, Zhang L, Luo C, Jiang M. Emerging strategies in cancer therapy combining chemotherapy with immunotherapy. Cancer Lett. 2019;454:191–203.
23. Galluzzi L, Buque A, Kepp O, Zitvogel L, Kroemer G. Immunological effects of conventional chemotherapy and targeted anticancer agents. Cancer Cell. 2015;28(6):690–714.
24. Janeway CA Jr., Goodnow CC, Danger MR. – pathogen on the premises! Immunological tolerance. Curr Biol. 1996;6(5):519–522.
25. Matzinger P. The danger model: a renewed sense of self. Science. 2002;296(5566):301–305.
26. Correale P, Aquino A, Giuliani A, et al. Treatment of colon and breast carcinoma cells with 5-fluorouracil enhances expression of carcinoembryonic antigen and susceptibility to HLA-A(*)02.01 restricted, CEA-peptide-specific cytotoxic T cells in vitro. Int J Cancer. 2003;104(4):437–445.
27. Wan S, Pestka S, Jubin RG, Lyu YL, Tsai YC, Liu LF. Chemotherapeutics and radiation stimulate MHC class I expression through elevated interferon-beta signaling in breast cancer cells. PLoS One. 2012;7(3):e32542.
28. Pol J, Vacchelli E, Aranda F, et al. Trial watch: immunogenic cell death inducers for anticancer chemotherapy. Oncoimmunology. 2015;4(4):e1008866.
29. Dudek AM, Garg AD, Krysko DV, De Ruysscher D, Agostinis P. Inducers of immunogenic cancer cell death. Cytokine Growth Factor Rev. 2013;24(4):319–333.
30. Zitvogel L, Kepp O, Senovilla L, Menger L, Chaput N, Kroemer G. Immunogenic tumor cell death for optimal anticancer therapy: the calreticulin exposure pathway. Clin Cancer Res. 2010;16(12):3100–3104.
31. Emens LA, Middleton G. The interplay of immunotherapy and chemotherapy: harnessing potential synergies. Cancer Immunol Res. 2015;3(5):436–443.
32. Ma Y, Adjemian S, Mattarollo SR, et al. Anticancer chemotherapy-induced intratumoral recruitment and differentiation of antigen-presenting cells. Immunity. 2013;38(4):729–741.
33. Martins I, Kepp O, Schlemmer F, et al. Restoration of the immunogenicity of cisplatin-induced cancer cell death by endoplasmic reticulum stress. Oncogene. 2011;30(10):1147–1158.
34. Galluzzi L, Humeau J, Buqué A, Zitvogel L, Kroemer G. Immunostimulation with chemotherapy in the era of immune checkpoint inhibitors. Nat Rev Clin Oncol. 2020;17(12):725–741.
35. Hodge JW, Garnett CT, Farsaci B, et al. Chemotherapy-induced immunogenic modulation of tumor cells enhances killing by cytotoxic T lymphocytes and is distinct from immunogenic cell death. Int J Cancer. 2013;133(3):624–636.
36. Tanaka H, Matsushima H, Mizumoto N, Takashima A. Classification of chemotherapeutic agents based on their differential in vitro effects on dendritic cells. Cancer Res. 2009;69(17):6978–6986.
37. Salem ML, Kadima AN, El-Naggar SA, et al. Defining the ability of cyclophosphamide preconditioning to enhance the antigen-specific CD8+ T-cell response to peptide vaccination: creation of a beneficial host microenvironment involving type I IFNs and myeloid cells. J Immunother. 2007;30(1):40–53.
38. Zhou S, Shang Q, Wang N, Li Q, Song A, Luan Y. Rational design of a minimalist nanoplatform to maximize immunotherapeutic efficacy: four birds with one stone. J Control Release. 2020;328:617–630.
39. Ren X, Wang N, Zhou Y, et al. An injectable hydrogel using an immunomodulating gelator for amplified tumor immunotherapy by blocking the arginase pathway. Acta Biomater. 2021;124:179–190.
40. DeNardo DG, Brennan DJ, Rexhepaj E, et al. Leukocyte complexity predicts breast cancer survival and functionally regulates response to chemotherapy. Cancer Discov. 2011;1(1):54–67.
41. Ge Y, Domschke C, Stoiber N, et al. Metronomic cyclophosphamide treatment in metastasized breast cancer patients: immunological effects and clinical outcome. Cancer Immunol Immunother. 2011;61(3):353–362.
42. Madondo MT, Quinn M, Plebanski M. Low dose cyclophosphamide: mechanisms of T cell modulation. Cancer Treat Rev. 2016;42:3–9.
43. Wesolowski R, Duggan MC, Stiff A, et al. Circulating myeloid-derived suppressor cells increase in patients undergoing neo-adjuvant chemotherapy for breast cancer. Cancer Immunol Immunother. 2017;66(11):1437–1447.
44. Alizadeh D, Trad M, Hanke NT, et al. Doxorubicin eliminates myeloid-derived suppressor cells and enhances the efficacy of adoptive T-cell transfer in breast cancer. Cancer Res. 2014;74(1):104–118.
45. Shree T, Olson OC, Elie BT, et al. Macrophages and cathepsin proteases blunt chemotherapeutic response in breast cancer. Genes Dev. 2011;25(23):2465–2479.
46. Chen DS, Mellman I. Oncology meets immunology: the cancer-immunity cycle. Immunity. 2013;39(1):1–10.
47. Murciano-Goroff YR, Warner AB, Wolchok JD. The future of cancer immunotherapy: microenvironment-targeting combinations. Cell Res. 2020;30(6):507–519.
48. Kwapisz D. Pembrolizumab and atezolizumab in triple-negative breast cancer. Cancer Immunol Immunother. 2021;70(3):607–617.
49. Jin Q, Liu Z, Chen Q. Controlled release of immunotherapeutics for enhanced cancer immunotherapy after local delivery. J Control Release. 2021;329:882–893.
50. Sharma P, Allison JP. The future of immune checkpoint therapy. Science. 2015;348(6230):56–61.
51. Pardoll DM. The blockade of immune checkpoints in cancer immunotherapy. Nat Rev Cancer. 2012;12(4):252–264.
52. Nanda R, Chow LQ, Dees EC, et al. Pembrolizumab in patients with advanced triple-negative breast cancer: phase Ib KEYNOTE-012 study. J Clin Oncol. 2016;34(21):2460–2467.
53. Adams S, Loi S, Toppmeyer D, et al. Pembrolizumab monotherapy for previously untreated, PD-L1-positive, metastatic triple-negative breast cancer: cohort B of the Phase II KEYNOTE-086 study. Ann Oncol. 2019;30(3):405–411.
54. Winer EP, Lipatov O, Im S-A, et al. Pembrolizumab versus investigator-choice chemotherapy for metastatic triple-negative breast cancer (KEYNOTE-119): a randomised, open-label, Phase 3 trial. Lancet Oncol. 2021;22(4):499–511.
55. Voorwerk L, Slagter M, Horlings HM, et al. Immune induction strategies in metastatic triple-negative breast cancer to enhance the sensitivity to PD-1 blockade: the TONIC trial. Nat Med. 2019;25(6):920–928.
56. Cortes J, Cescon DW, Rugo HS, et al. Pembrolizumab plus chemotherapy versus placebo plus chemotherapy for previously untreated locally recurrent inoperable or metastatic triple-negative breast cancer (KEYNOTE-355): a randomised, placebo-controlled, double-blind, phase 3 clinical trial. Lancet. 2020;396(10265):1817–1828.
57. Dill EA, Dillon PM, Bullock TN, Mills AM. IDO expression in breast cancer: an assessment of 281 primary and metastatic cases with comparison to PD-L1. Mod Pathol. 2018;31(10):1513–1522.
58. Muller AJ, DuHadaway JB, Donover PS, Sutanto-Ward E, Prendergast GC. Inhibition of indoleamine 2,3-dioxygenase, an immunoregulatory target of the cancer suppression gene Bin1, potentiates cancer chemotherapy. Nat Med. 2005;11(3):312–319.
59. Lu J, Liu X, Liao YP, et al. Breast cancer chemo-immunotherapy through liposomal delivery of an immunogenic cell death stimulus plus interference in the IDO-1 pathway. ACS Nano. 2018;12(11):11041–11061.
60. Keane MM, Ettenberg SA, Nau MM, Russell EK, Lipkowitz S. Chemotherapy augments TRAIL-induced apoptosis in breast cell lines. Cancer Res. 1999;59(3):734–741.
61. Bhola NE, Balko JM, Dugger TC, et al. TGF-beta inhibition enhances chemotherapy action against triple-negative breast cancer. J Clin Invest. 2013;123(3):1348–1358.
62. Zhou M, Luo C, Zhou Z, Li L, Huang Y. Improving anti-PD-L1 therapy in triple negative breast cancer by polymer-enhanced immunogenic cell death and CXCR4 blockade. J Control Release. 2021;334:248–262.
63. Roy A, Singh MS, Upadhyay P, Bhaskar S. Nanoparticle mediated co-delivery of paclitaxel and a TLR-4 agonist results in tumor regression and enhanced immune response in the tumor microenvironment of a mouse model. Int J Pharm. 2013;445(1–2):171–180.
64. Vicari AP, Luu R, Zhang N, et al. Paclitaxel reduces regulatory T cell numbers and inhibitory function and enhances the anti-tumor effects of the TLR9 agonist PF-3512676 in the mouse. Cancer Immunol Immunother. 2009;58(4):615–628.
65. Seth A, Heo MB, Lim YT. Poly (gamma-glutamic acid) based combination of water-insoluble paclitaxel and TLR7 agonist for chemo-immunotherapy. Biomaterials. 2014;35(27):7992–8001.
66. Vacchelli E, Galluzzi L, Eggermont A, et al. Trial watch: FDA-approved Toll-like receptor agonists for cancer therapy. Oncoimmunology. 2012;1(6):894–907.
67. Le Naour J, Zitvogel L, Galluzzi L, Vacchelli E, Kroemer G. Trial watch: STING agonists in cancer therapy. Oncoimmunology. 2020;9(1):1777624.
68. Gao S, Yang X, Xu J, Qiu N, Zhai G. Nanotechnology for boosting cancer immunotherapy and remodeling tumor microenvironment: the horizons in cancer treatment. ACS Nano. 2021.
69. Gu X, Gao Y, Wang P, et al. Nano-delivery systems focused on tumor microenvironment regulation and biomimetic strategies for treatment of breast cancer metastasis. J Control Release. 2021;333:374–390.
70. Du B, Wang Q, Yang Y, et al. Two-way cruise nanosatellite promotes metastasis inhibition by immunochemotherapy. Biomacromolecules. 2019;20(7):2873–2887.
71. Mei KC, Liao YP, Jiang J, et al. Liposomal delivery of mitoxantrone and a cholesteryl indoximod prodrug provides effective chemo-immunotherapy in multiple solid tumors. ACS Nano. 2020;14(10):13343–13366.
72. Jiang T, Mo R, Bellotti A, Zhou J, Gu Z. Gel-liposome-mediated co-delivery of anticancer membrane-associated proteins and small-molecule drugs for enhanced therapeutic efficacy. Adv Funct Mater. 2014;24(16):2295–2304.
73. Chen M, Miao Y, Qian K, et al. Detachable liposomes combined immunochemotherapy for enhanced triple-negative breast cancer treatment through reprogramming of tumor-associated macrophages. Nano Lett. 2021;21(14):6031–6041.
74. Chen C, Shen M, Liao H, et al. A paclitaxel and microRNA-124 coloaded stepped cleavable nanosystem against triple negative breast cancer. J Nanobiotechnology. 2021;19:1.
75. Lan Y, Liang Q, Sun Y, et al. Codelivered chemotherapeutic doxorubicin via a dual-functional immunostimulatory polymeric prodrug for breast cancer immunochemotherapy. ACS Appl Mater Interfaces. 2020;12(28):31904–31921.
76. Lee AL, Wang Y, Pervaiz S, Fan W, Yang YY. Synergistic anticancer effects achieved by co-delivery of TRAIL and paclitaxel using cationic polymeric micelles. Macromol Biosci. 2011;11(2):296–307.
77. Qin T, Xu X, Zhang Z, et al. Paclitaxel/sunitinib-loaded micelles promote an antitumor response in vitro through synergistic immunogenic cell death for triple-negative breast cancer. Nanotechnology. 2020;31(36):365101.
78. Hernandez-Gil J, Cobaleda-Siles M, Zabaleta A, Salassa L, Calvo J, Mareque-Rivas JC. An iron oxide nanocarrier loaded with a Pt(IV) prodrug and immunostimulatory dsRNA for combining complementary cancer killing effects. Adv Healthc Mater. 2015;4(7):1034–1042.
79. Zhu Y, Yang Z, Dong Z, et al. CaCO3-assisted preparation of pH-responsive immune-modulating nanoparticles for augmented chemo-immunotherapy. Nanomicro Lett. 2020;13(1):29.
80. Liu Y, Qiao L, Zhang S, et al. Dual pH-responsive multifunctional nanoparticles for targeted treatment of breast cancer by combining immunotherapy and chemotherapy. Acta Biomater. 2018;66:310–324.
81. Wang L, Ding K, Zheng C, et al. Detachable nanoparticle-enhanced chemoimmunotherapy based on precise killing of tumor seeds and normalizing the growing soil strategy. Nano Lett. 2020;20(9):6272–6280.
82. Wang Y, Gao D, Liu Y, et al. Immunogenic-cell-killing and immunosuppression-inhibiting nanomedicine. Bioact Mater. 2021;6(6):1513–1527.
83. Zhang J, Zhang Y, Zhao B, et al. Cascade-responsive hierarchical nanosystems for multisite specific drug exposure and boosted chemoimmunotherapy. ACS Appl Mater Interfaces. 2021;13(49):58319–58328.
84. Hu Q, Shang L, Wang M, et al. Co-delivery of paclitaxel and interleukin-12 regulating tumor microenvironment for cancer immunochemotherapy. Adv Healthc Mater. 2020;9(10):e1901858.
85. Shen S, Li HJ, Chen KG, et al. Spatial targeting of tumor-associated macrophages and tumor cells with a pH-sensitive cluster nanocarrier for cancer chemoimmunotherapy. Nano Lett. 2017;17(6):3822–3829.
86. Liang J, Wang H, Ding W, et al. Nanoparticle-enhanced chemo-immunotherapy to trigger robust antitumor immunity. Sci Adv. 2020;6(35):eabc3646.
87. Wang C, Wang J, Zhang X, et al. In situ formed reactive oxygen species-responsive scaffold with gemcitabine and checkpoint inhibitor for combination therapy. Sci Transl Med. 2018;10:429.
88. Wang Z, Zhang Y, Liu Z, et al. A bifunctional nanomodulator for boosting CpG-mediated cancer immunotherapy. Nanoscale. 2017;9(37):14236–14247.
89. Zhou M, Wang X, Lin S, et al. Multifunctional STING-activating Mn3 O4 @Au-dsDNA/DOX nanoparticle for antitumor immunotherapy. Adv Healthc Mater. 2020;9(13):e2000064.
90. Chen L, Zhou L, Wang C, et al. Tumor-targeted drug and CpG delivery system for phototherapy and docetaxel-enhanced immunotherapy with polarization toward M1-type macrophages on triple negative breast cancers. Adv Mater. 2019;31(52):e1904997.
91. Zheng X, Zhao Y, Jia Y, et al. Biomimetic co-assembled nanodrug of doxorubicin and berberine suppresses chemotherapy-exacerbated breast cancer metastasis. Biomaterials. 2021;271:120716.
92. Du W, Chen C, Sun P, et al. Eliciting an immune hot tumor niche with biomimetic drug-based multi-functional nanohybrids augments immune checkpoint blockade-based breast cancer therapy. Nanoscale. 2020;12(5):3317–3329.
93. Wu T, Qiao Q, Qin X, Zhang D, Zhang Z. Immunostimulatory cytokine and doxorubicin co-loaded nanovesicles for cancer immunochemotherapy. Nanomedicine. 2019;18:66–77.
94. Lonez C, Vandenbranden M, Ruysschaert JM. Cationic liposomal lipids: from gene carriers to cell signaling. Prog Lipid Res. 2008;47(5):340–347.
95. Deng C, Zhang Q, Jia M, et al. Tumors and their microenvironment dual-targeting chemotherapy with local immune adjuvant therapy for effective antitumor immunity against breast cancer. Adv Sci. 2019;6(6):1801868.
96. Liu J, Ai X, Cabral H, Liu J, Huang Y, Mi P. Tumor hypoxia-activated combinatorial nanomedicine triggers systemic antitumor immunity to effectively eradicate advanced breast cancer. Biomaterials. 2021;273:120847.
97. Nicolas J, Mura S, Brambilla D, Mackiewicz N, Couvreur P. Design, functionalization strategies and biomedical applications of targeted biodegradable/biocompatible polymer-based nanocarriers for drug delivery. Chem Soc Rev. 2013;42(3):1147–1235.
98. Jin H, Wan C, Zou Z, et al. Tumor ablation and therapeutic immunity induction by an injectable peptide hydrogel. ACS Nano. 2018;12(4):3295–3310.
99. Dong X, Yang A, Bai Y, Kong D, Lv F. Dual fluorescence imaging-guided programmed delivery of doxorubicin and CpG nanoparticles to modulate tumor microenvironment for effective chemo-immunotherapy. Biomaterials. 2020;230:119659.
100. Song Q, Yin Y, Shang L, et al. Tumor microenvironment responsive nanogel for the combinatorial antitumor effect of chemotherapy and immunotherapy. Nano Lett. 2017;17(10):6366–6375.
101. Oliva N, Conde J, Wang K, Artzi N. Designing hydrogels for on-demand therapy. Acc Chem Res. 2017;50(4):669–679.
102. Yang X, Zhao M, Wu Z, et al. Nano-ultrasonic contrast agent for chemoimmunotherapy of breast cancer by immune metabolism reprogramming and tumor autophagy. ACS Nano. 2022;16(2):3417–3431.
103. Zheng DW, Chen JL, Zhu JY, et al. Highly integrated nano-platform for breaking the barrier between chemotherapy and immunotherapy. Nano Lett. 2016;16(7):4341–4347.
104. Zhang F, Chen F, Yang C, et al. Coordination and redox dual-responsive mesoporous organosilica nanoparticles amplify immunogenic cell death for cancer chemoimmunotherapy. Small. 2021;17(26):e2100006.
105. Cheng Y, Chen Q, Guo Z, et al. An intelligent biomimetic nanoplatform for holistic treatment of metastatic triple-negative breast cancer via photothermal ablation and immune remodeling. ACS Nano. 2020;14(11):15161–15181.
106. Hu M, Zhang J, Kong L, et al. Immunogenic hybrid nanovesicles of liposomes and tumor-derived nanovesicles for cancer immunochemotherapy. ACS Nano. 2021;15(2):3123–3138.
107. Li S, Wang Q, Shen Y, et al. Pseudoneutrophil cytokine sponges disrupt myeloid expansion and tumor trafficking to improve cancer immunotherapy. Nano Lett. 2020;20(1):242–251.
108. Syn NL, Wang L, Chow EK, Lim CT, Goh BC. Exosomes in cancer nanomedicine and immunotherapy: prospects and challenges. Trends Biotechnol. 2017;35(7):665–676.
109. Wang P, Wang H, Huang Q, et al. Exosomes from M1-polarized macrophages enhance paclitaxel antitumor activity by activating macrophages-mediated inflammation. Theranostics. 2019;9(6):1714–1727.