Applications of Carbon Dots for the Treatment of Alzheimer’s Disease
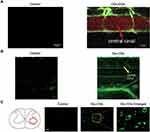
Introduction
Alzheimer’s dementia is one of the greatest global challenges for health and social care in the 21st century. Approximately 50 million people worldwide currently suffer from Alzheimer’s disease, and the number is expected to triple by 2050. While there are many different illnesses that can cause dementia, Alzheimer’s disease (AD) is the most common.1,2 AD results in a progressive deterioration of brain function, initially characterized by cognitive deficits, with loss of recent memory and language ability, impairment of orientation, problem solving, and abstract thinking.3
The pathophysiology of AD is very complex, but has the primary histopathological features in the brain of extracellular plaques comprised of amyloid β (Aβ) and intracellular neurofibrillary tangles (NFTs) comprised of hyperphosphorylated tau protein.4–6 Although great progress has been made in exploring the etiology of AD, the exact pathogenesis of the disease is still unclear.7 Since patients with more advanced disease require full-time care, AD imposes a heavy burden on both families and society.8 Currently there are two classes of drugs that are most used for the treatment of AD: acetylcholinesterase inhibitors, which act to boost brain levels of acetylcholine, a neurotransmitter known to play an important role in cognition,9–13 and an antagonist to the glutamatergic N-methyl d-aspartate (NMDA) receptor, which seem to act by preventing overactivation of excitatory neurotransmission.14 Very recently, a third approach to the treatment of AD was approved for use in the United States. The drug, aducanumab, is a monoclonal antibody that acts to remove amyloid from the brain. However, currently available clinical data indicate that the drug is, at best, only marginally effective, fails to protect patients from cognitive and functional decline, and carries significant risks including brain swelling.15 Thus, currently available treatments for AD have limited efficacy, marginally slowing symptom progression in AD patients, and cannot prevent the progress of brain damage, or cure the disease.13–16
With the exception of aducanumab, drug treatments for AD are administered orally. Oral administration is convenient for patients, but presents many obstacles to achieving desired effects, including poor bioavailability, low absorption, first-pass metabolism, and dose-dependent side effects.12 When targeting the brain, presence of the blood-brain barrier (BBB) is an additional problem. The BBB is a unique and complex multicellular structural barrier separating the central nervous system from the rest of the body, composed of a semi-permeable membrane of endothelial cells.17 The BBB prevents viruses and most macromolecules from entering the CNS, shields the brain from blood-borne pathogens and other hazardous chemicals, and is the human body’s most tightly regulated biological barrier.18 However, these clearly beneficial functions of the BBB are countered by the difficulty it creates for delivering many potentially therapeutic compounds to the brain.
One promising method to overcome BBB is to load drugs into nanocontainers, which are characterized by relatively small size and the ability to cross the BBB.19–22 Using this drug delivery method can significantly improve the bioavailability of therapeutic substances and reduce side effects.12 Nano-formulations are characterized by their plasticity and modified surface properties, flexibility and controlled release of active drug substances, which can target CNS active drugs to the brain through the BBB.23 Studies also reveal that nanotechnological approaches can aid in early diagnosis of AD and enhance therapeutic efficacy, and have demonstrated tremendous potential for the development of effective brain-targeted therapeutics.6,24 Carbon dots (CDs) are one of the most promising and novel nanocarriers with potential nanomedicine applications25,26 because they are relatively easy to synthesize, and have advantageous properties such as ultra-small size, good photostability, outstanding biocompatibility, tunable photoluminescence (PL), chemical stability and low cytotoxicity.27 In addition, CDs have clear potential as an advanced drug delivery system with the capacity to cross BBB.28,29 CDs are usually spherical or hemispherical, with a size of less than 10 nm, and are mainly composed of a core of sp2 inter-hybridized carbon atoms. The surface has many functional groups,30 giving CDs a rich morphology and structure and a large number of surface groups/polymer chains such as carboxyl, hydroxyl, amine, etc., that promote water solubility, which is convenient for compounding with other materials without phase separation.31,32 In addition, abundant functional groups make CDs easily modified. While we use the general term “carbon dots” in this review, classes of CDs can be subdivided into carbon quantum dots (CQDs), graphene quantum dots (GQDs), carbon nanodots (CNDs) and carbonized polymer dots (CPDs) according to their carbon core structure, surface functional groups, and their properties.33,34 Carbon sources include inexpensive and low-toxicity molecules such as citric acid,35 ethanol,36 glycerol,37 flour38 and even plant tissues.39 The aim of the current review is to provide a summary of current knowledge concerning the development and potential applications of using CDs for the diagnosis and treatment of Alzheimer’s disease.
CD Characteristics, Synthesis, Safety and Ability to Cross the BBB
CDs can have different sizes and multifunctional surface moieties to facilitate the loading and delivery of drug molecules across the BBB. As reported by Zhang et al,40 after combining of folic acid (FA) and CDs, the photostable FA-CDs can selectively enter HepG2 cancer cells through folate receptor (FR)-mediated endocytosis. However, depending on the size and surface dopants, the transport mechanism differs. Most CDs, because of their small size, polymer core structure or various functional groups on the surface,41,42 promote the delivery of macromolecular drugs through carrier-mediated transport covalently bound to the drug.43–45 However, in order to improve transport efficiency, multiple mechanisms can be engaged to increase the ability of CDs to traverse the BBB by 50–100%.46 At the same time, PL characteristics of CDs can be used to track the penetration of CDs through BBB.47
The production methods of CDs can generally be divided into “top-down” and “bottom-up” approaches, using carbon materials or organic matter as precursors, respectively.48–52 The “top-down” method generates CDs by oxidizing double bonds on macromolecular starting materials such as coarse carbon powder53 via arc discharge,48 laser ablation54–56 or electrochemical oxidation.52,57,58 The “bottom-up” method uses small organic molecules to synthesize CDs. The “bottom-up” approach involves polymerization of small monomer units (such as citric acid or amines) through covalent and hydrogen bonding28 achieved through combustion,59 thermal carbonization,51 acid dehydration or ultrasonic treatment.37 Reaction time, solvent and temperature are manipulated to change the chemical structure and size of CDs.60–65 The “bottom-up” synthesis method is usually more flexible and environmentally friendly, and its use is more common.46 Table 1 summarizes several classic synthesis methods and relevant characteristics of the resultant CDs.
![]() |
Table 1 Characteristics of Carbon Dots Generated Through Different Synthetic Methods |
As is shown in Table 1, CDs obtained by different synthesis methods show different emission peak positions.63,72–74 Most CDs show strong absorption peaks in the ultraviolet region, and the formation of absorption peaks/shoulder peaks is attributed to π– π* (C=C bond), n–π* transition (C=O bond) or other.63,73 It is worth noting that changes in oxygen/nitrogen content, structural defects and CD morphology play a key role in determining the position of absorption peaks.50
Systemic administration of CDs appears to be very safe. Although CDs can be subsequently found in various organs, the accumulation is very low. No significant signs of toxicity have been reported, such as clinical symptoms, death, or significant weight loss.75 In addition, under the high concentration of CDs required for PL bioimaging, histopathological results from treated mice did not show obvious damage.76 Intravenously delivered CDs are mainly excreted through urine,77 a method of elimination of PEGylated nanoparticles widely reported in the literature, especially for the use of very small particles.78 Ex vivo imaging analysis revealed that only dissected kidney and liver showed meaningful fluorescence from CDs; the former was brighter, consistent with the urinary excretion pathway. Different injection methods affect the biodistribution of CDs in major organs and tissues as well as the clearance rate. The clearance rate of CDs in descending order is intravenous (iv) > intramuscular (im) > subcutaneous (sc).79
Importantly in the current context, the plasticity and modified surface properties of nanoforulations allow them to carry neuroactive drugs across the BBB for targeted delivery to the brain. In addition, the surface characteristics of CDs can be modified to facilitate the process, such as binding to transferrin as a means of transporting neuroactive substances into the brain.80,81 Li et al used carbon powder as a raw material to synthesize CDs using a “top-down” method, and achieved effective Dox delivery through receptor-mediated endocytosis.82 In the same year, Li et al83 used zebrafish as a biological model to study whether CDs coupled to transferrin can cross the BBB. They found that CDs alone could not overcome the BBB, but that transferrin-CDs were able to do so through transferrin-receptor-mediated endocytosis.
It is also possible to cross the BBB through transport proteins present on the cell membrane surface. Mintz et al84 used tryptophan as a carbon precursor, mixed with 1,2-ethylenediamine (EDA) and urea, respectively, with two N-dopants, to obtain two kinds of products. The substances in the yellow gel state, namely CDs-EDA and CDs-urea, appeared to have tryptophan moieties on the surface, and exhibited bright photoluminescence (QY=48%) and low toxicity. Most prominently, CDs-EDA were shown to cross the BBB and enter the CNS of zebrafish through endocytosis mediated by the LAT1 transporter (Figure 1A). The two CDs demonstrated the possibility of delivering drugs to the CNS without binding to receptors. More recently, Seven et al85 used glucose as the substrate to synthesize GluCDs to take advantage of a glucose transporter to deliver CDs across the BBB. Importantly, this study demonstrated that a non-receptor mediated transport mechanism could be used to deliver CDs to the central nervous system of a mammal following intravenous administration. Examples of CNS penetration of Glu-CDs in zebrafish and rat models are shown in Figures 1B and C, respectively.
![]() |
Figure 1 Examples of central nervous system penetration by carbon dots designed to use transporters to cross the blood-brain barrier. (A) CDs-EDA with attached tryptophan moieties appear in the central canal of zebrafish spinal cord following injection into the heart. Spinal cord is labeled with cherry red; central canal appears as a thin yellow band at base of red arrow. ((A) Adapted with permission from Colloids Surf B Biointerfaces, Vol 176, Mintz KJ, Mercado G, Zhou Y, et al, Tryptophan carbon dots and their ability to cross the blood-brain barrier, Pages 488–493, Copyright 2019, with permission from Elsevier.84 (B) Glu-CDs appear in zebrafish central canal (thin green line at head of yellow arrow) following injection into the heart. (C) After Glu-CDs injection into rat tail vein, labeled neurons are seen in neurons in spinal cord gray matter. (B and C) Adapted from Seven ES, Seven YB, Zhou Y, et al. Crossing the blood-brain barrier with carbon dots: uptake mechanism and in vivo cargo delivery. Nanoscale Adv. 2021;3(13):3942–3953.85 2021, with permission from the Royal Society of Chemistry. |
The hydrophilicity and hydrophobicity of CDs can also be adjusted so that they can cross the BBB by passive diffusion. For example, Zhou et al25 created novel amphiphilic nontoxic CDs (Y-CDs), synthesized by an ultrasound-mediated method, with small size (3.4 ± 1.0 nm) and low zeta potential (−15.3 mV). Y-CDs exhibit solvent effects, which suggests that Y-CDs can be dispersed in many different organic solvents and exhibit different PL properties. Therefore, Y-CDs have amphiphilic properties and can mediate drug delivery via passive diffusion. However, one of the most attractive features of Y-CDs is that this amphiphilicity is not altered by conjugation to hydrophilic molecules. Under excitation at 405 nm, yellow fluorescence from Y-CDs could be clearly observed in the central canal of zebrafish spinal cord following intracardial injection, indicating that Y-CDs crossed the BBB. This is illustrated in Figure 2.
![]() |
Figure 2 Y-CDs appear in zebrafish spinal cord central canal follow injection into the heart. Central canal is thin yellow line at the head of the red arrow. Used with permission of Royal Society of Chemistry, from Zhou Y, Liyanage PY, Devadoss D, et al. Nontoxic amphiphilic carbon dots as promising drug nanocarriers across the blood-brain barrier and inhibitors of β-amyloid. Nanoscale. 2019;11(46):22387–22397; permission conveyed through Copyright Clearance Center, Inc.25 |
Potential Applications of Carbon Dots to the Treatment of AD
Solutions Targeting Amyloid to Reduce AD Pathology
Many studies to date suggest promising applications for CDs in the treatment of AD. Because of the association of amyloid plaques with AD, they have long been the target of potential therapeutic approaches. Unfortunately, the many positive results in preclinical studies have yet to translate into effective therapies in humans. Nevertheless, a significant amount of research targeting amyloid continues, and it may be that modifying drug delivery systems that affect amyloid synthesis or aggregation could be a critical for the success of this approach. Han et al86 were the first to explore the effects of CDs on Aβ. They found that CDs inhibited in vitro Aβ fibrillation of both Aβ40 and Aβ42. In addition, CDs were found to inhibit the activity of β-site amyloid precursor protein cleaving enzyme 1 (BACE1), a key enzyme for generating these Aβ fragments. The authors also demonstrated that CDs inhibited the negative effects of Aβ42 fibrils on the development of sea urchin embryos, likely by delaying the formation of Aβ42 toxic species. Finally, the authors confirmed the earlier findings of Li et al83 that CDs conjugated to transferrin would cross the BBB to localize in the brain of a zebrafish model, while retaining their inhibitory activity against Aβ fibrillation and BACE1 activity.
As mentioned above, Zhou et al25 synthesized Y-CDs that were shown to cross the BBB and exhibited good nanocarrier properties. In addition, the authors found that, using in vitro studies, Y-CDs reduced the expression and secretion of Aβ by inhibiting the production of amyloid precursor protein (APP). As mentioned earlier, Y-CDs exhibit amphiphilicity, which means that Y-CDs not only have a hydrophilic surface but also exhibit more hydrophobic functions than hydrophilic CDs. The hydrophilic surface can interact with Aβ alone to form more extended structures, making it more flexible, and the increased flexibility will be beneficial for inhibiting Aβ fibril formation.87,88 Importantly, Zhou et al examined the toxicity of Y-CDs in four different cell lines and demonstrated that Y-CDs were nontoxic at the concentrations tested. More recently, Yan et al89 combined Y-CDs with Ce6, a photosensitizer, and showed that this combination crossed the BBB after tail vein injections in mice. Further the Y-CD/Ce6 combination reduced amyloid aggregation and toxicity, and removed amyloid plaques from the brains of the APP/PS1 Alzheimer’s disease mouse model.
It has been convincing shown that copper ions increase the risk for, and magnitude of, AD pathogenesis.90,91 The Cu(II)-bound Aβ complex (Aβ-Cu(II)) is one of the most toxic Aβ species known.92 In addition, Cu(II) with redox activity triggers oxidative stress in Fenton-type reactions and increases Aβ-mediated cytotoxicity.93 Chung et al94 designed multifunctional CDs that can chelate Cu(II) ions, inhibit Aβ aggregation and photo oxidize Aβ peptides. These investigators conjugated CDs and Aβ-Cu(II) by using o-phenylenediamine (OPD) as a polymerization precursor to generate pOPD-CDs. The nucleophilic amine and imine groups on the surface of these CDs exhibit high affinity for cationic metal ions such as Cu(II). Synthetic pOPD-CDs exhibit a variety of anti-AD properties. Their surface attracts cationic Cu(II) and interrupts the orderly β-sheet accumulation of Aβ peptides. At the same time, under light irradiation, light-excited pOPD-CDs can further oxidize Aβ residues, leading to light enhancement and inhibition of Cu(II)-mediated Aβ aggregation (Figure 3).
![]() |
Figure 3 (A) The MALDI-TOF mass spectra of Aβ-Cu(II) aggregates incubated with pOPD-CDs for 24 hours at 37°C. Light was irradiated only for the initial one hour during incubation. (B) Atomic force microscopy (AFM) images, and (C) spectra of Aβ-Cu(II) aggregates incubated with or without pOPD-CDs under dark and one-hour-light illuminating conditions (scale bar: 1μm). The concentration used in all samples was 50μg/mL. For light irradiation, a blue LED was used. Used with permission of Royal Society of Chemistry, from Chung YJ, Lee BI, Park CB. Multifunctional carbon dots as a therapeutic nanoagent for modulating Cu(ii)-mediated β-amyloid aggregation. Nanoscale. 2019;11(13):6297–6306; permission conveyed through Copyright Clearance Center, Inc.94 |
In later work, Chung et al95 used a novel approach to disrupt β-amyloid aggregation. They used citric acid and urea as precursors to synthesize red light-absorbing CDs (RCDs) under the solvothermal conditions of an aprotic solvent, and then mixed the RCDs with a DNA aptamer sequence to Aβ peptides. These CDs, termed [email protected], showed good Aβ species targeting, co-localizing with Aβ aggregates in brain tissue in vitro and in vivo following injection into the brain of transgenic 5xFAD mice, a model of AD. In addition, [email protected] under red light effectively inhibited the formation of the Aβ aggregates rich in neurotoxic β-sheets by oxidizing adjacent Aβ species. Importantly, unlike the original Aβ peptide, the light-modulated Aβ species had negligible toxicity to neuronal PC12 cells. Chung et al’s findings demonstrated the therapeutic potential of light-regulated CDs for inhibiting Aβ self-assembly and showed light-driven suppression of associated neurotoxicity, establishing another potential new platform and path for the treatment of AD. As the authors note, the next steps in this work will be to insure that [email protected] can be delivered to the brain following systemic administration and to develop minimally invasive methods for their red-light activation.
Curcumin (CUR) is a small polyphenol molecule extracted from turmeric.96 CUR has anticancer, anti-inflammatory, antioxidant, antibacterial, and neuroprotective effects97 and may prevent or ameliorate the pathological processes underlying age-related cognitive decline, AD, or other dementias.98 There is increasing evidence that CUR may partially protect cells from Aβ-mediated neurotoxicity by inhibiting Aβ aggregation.99 Zheng et al100 explored whether CUR could reduce memory decline by inhibiting BACE1 expression and β-amyloid pathology in 5×FAD transgenic mice. It was found that CUR reduced amyloid plaque deposition and soluble Aβ1-42 in the brain by inhibiting BACE1. In addition, CUR was found to upregulate synaptophysin expression (essential for maintaining synaptic transmission)102,103 and attenuate synaptic damage in 5×FAD mice. Finally, learning and memory in CUR-treated mice were improved.
Although CUR shows promise for clinical treatment of diseases97, its poor water solubility and water stability result in very low cell-targeted absorption efficiency and bioavailability, and can cause significant cytotoxicity at high concentrations.99 To better improve the cell uptake efficiency and bioavailability of CUR, Kuang et al99 mixed citric acid and glucose to synthesize CDs using a microwave method. They then combined the as-prepared CDs with CH3COONa and FeCl3 to obtain Fe3O4@CDs, which were subsequently combined with CUR. In vitro studies showed that CUR-Fe3O4@CDs had a high specific affinity for Aβ42 and could significantly inhibit the aggregation of Aβ42 protein. The [email protected] also inhibited the production of reactive oxygen species (ROS) mediated by Aβ fibrils and reduced Aβ-mediated toxicity in PC12 cells. These experiments all suggest that CDs could provide an efficient delivery system for CUR and thus a promising platform for AD therapy. However, studies are needed to demonstrate that CUR-Fe3O4@CDs cross the BBB and show in vivo efficacy.
Graphene Quantum Dots (GQDs) are a class of carbon dots synthesized from small fragments of graphene, and thus tend to have a planar instead of a spherical structure.101 GQDs have been shown to inhibit the aggregation of Aβ42 peptides in vitro,102 with the degree of inhibition proportional to the amount of surface negative charge. This study also showed that GQDs were able to significantly reduce the toxicity of Aβ1-42 in an in vitro PC12 cell model, while having negligible effects on cell viability themselves.
Xiao et al103 conjugated a neuroprotective peptide, glycine-proline-glutamate, to GQDs to generate GQDGs. These conjugated nanoparticles were shown to inhibit the aggregation of Aβ in vitro. Importantly, intravenous administration of GQCDs to transgenic APP/PS1 mice, another model of Alzheimer’s disease, reduced brain levels of Aβ, reduced brain inflammatory markers, increased brain neurotrophin levels and reduced learning and memory impairments in the mice (Figure 4A). No histopathological abnormalities were seen with the 4-week administration period. GQDGs also enhanced neurogenesis and improved dendritic morphology (Figure 4B) in the hippocampus (a brain structure known to be essential for many types of learning and memory) of treated mice.
![]() |
Figure 4 (A) After 4 weeks of intravenous administration of GQCDs to APP/PS1 mice, spatial learning in a water maze was significantly improved and approached that of wild-type controls. (B) Dendritic spine densities were markedly reduced in untreated or vehicle-treated APP/PS1 mice (TgCtrl or PBS, respectively). Treatment with GQCDs restored spine densities to near normal levels. Scale bars: 40µm, left; 10µm, right. Asterisks indicate **p < 0.01, ***p < 0.001 versus Tg Ctrl or PBS groups. Reprinted from Biomaterials, Vol 106, Xiao S, Zhou D, Luan P, et al, Graphene quantum dots conjugated neuroprotective peptide improve learning and memory capability, Pages 98–110, Copyright (2016), with permission from Elsevier.103 |
In a study published three years after their initial exploration of the effect of GQDs on Aβ aggregation, Liu et al104 covalently bound tramiprosate, a compound shown to inhibit amyloid aggregation that has shown some success in clinical trials with AD patients (Manzano et al)105, to GQDs. The resultant GQD-Ts showed a synergistic effect on inhibiting amyloid aggregation and fibril formation, while retaining the non-toxic nature of GQDs alone. It remains to be shown that GQD-Ts can penetrate the BBB and have in vivo efficacy. Table 2 is a summary of studies on the beneficial effects of CDs on amyloid pathology in recent years.
![]() |
Table 2 A Summary of Studies Demonstrating Beneficial Effects of CDs on Amyloid Pathology |
Solutions Targeting Tau to Improve AD Pathology
It is well known that tau plays an important role in maintaining the stability of cellular structures.11,107 In AD, hyperphosphorylation of tau disrupts the balance between tau phosphorylation and dephosphorylation, resulting in the production of a large amount of free ptau, which can dissociate from microtubules and self-aggregate to form NFTs in the cell body and dystrophic neurites, thereby destroying microtubule function. This eventually leads to neuronal death, a hallmark of AD.7,108 Current studies have proposed that preventing tau pathology could be a better target than amyloid for treating AD.109,110
In recent work, Zhang et al111 and Zhou et al112 investigated the ability of carbon nitride dots (CNDs) to inhibit tau aggregation. Further, they successfully combined CNDs with memantine hydrochloride, a drug long used to treat AD but with limited efficacy. It was found that both CNDs alone CNDs-MH exhibited the ability to inhibit tau aggregation in vitro (Figure 5). Further, these authors have shown that both CNDs and CNDs-MH can cross the BBB in a zebrafish model.111,113 Thus, this work provides a foundation for further studies to attack AD-related brain neuropathology via multiple mechanisms.
![]() |
Figure 5 Inhibition of microtubule associated protein tau (MAPT) aggregation using an in vitro fluorescence assay. (A) and (B): CNDs alone significantly inhibited tau aggregation at concentrations of 14.3 µg/mL and above. Memantine hydrochloride-conjugated CNDs (CNDs-MH) were equally effective in inhibiting tau aggregation. (C) and (D) Both CNDs and CNDs-MH were shown to cross the BBB in a zebrafish model (see text for references).Reprinted from Journal of Colloid and Interface Science, Vol 617, Zhang W, Kandel N, Zhou Y, et al, Drug delivery of memantine with carbon dots for Alzheimer’s disease: blood–brain barrier penetration and inhibition of tau aggregation, Pages 20–31, Copyright (2022), with permission from Elsevier.111 |
Solutions Targeting Acetylcholine to Improve AD Symptoms
As has been described, inhibition of acetylcholinesterase (AChE) to elevate levels of acetylcholine (Ach) in the brain is one of the few currently available symptomatic therapies for AD. Extracts of the leguminous butterfly pea can increase of Ach levels in the hippocampus, which can lead to improved learning and memory.114 Based upon this information, Tak et al115 used a one-pot microwave-assisted green synthesis method to synthesize GQDs (ctGQDs) from butterfly pea flowers. In an in vitro assay, ctGQDs were shown to inhibit AChE as effectively as donepezil, the most commonly used drug currently used to treat AD. Systemically administered ctGQDs were also shown to reverse the learning and memory deficits in rats induced by scopolamine, a cholinergic receptor antagonist. These results provide further evidence and GQDs are able to cross the BBB to affect brain function.
More recently, Suner et al71 used citric acid as a carbon source and cysteine (Cys) as a source of sulfur and nitrogen, and prepared S- and N-doped Cys CDs through a green synthesis technique using a microwave method. Their study showed that inhibition of AChE was proportional to the concentration of Cys CDs (Figure 6). Of course, it is not known whether this route of enzymatic inhibition would show better functional efficacy or a reduced side effect profile than existing pharmacotherapies, but the work clearly reinforces the idea that CDs can serve as an effective way of delivering agents to the brain.
![]() |
Figure 6 Inhibition of AChE enzyme activity by carbon dots. (A) Cys CD inhibition of AChE in vitro is proportional to carbon dot concentration. ((A) Reprinted by permission from Springer Nature Customer Service Centre GmbH: Springer Nature; Journal of Fluorescense; Versatile fluorescent carbon dots from citric acid and cysteine with antimicrobial, anti-biofilm, antioxidant, and AChE enzyme inhibition capabilitie, Suner SS, Sahiner M, Ayyala RS, et al, COPYRIGHT (2021).71 (B) ctGQDs inhibit AChE in rat hippocampus as effectively as Donepezil, a drug commonly prescribed to patients with Alzheimer’s disease. Asterisks indicate ***p < 0.001 versus no treatment. ((B) Adapted with permission from Tak K, Sharma R, Dave V, et al. Clitoria ternatea mediated synthesis of graphene quantum dots for the treatment of Alzheimer’s disease. ACS Chem Neurosci. 2020;11(22):3741–3748.115 © 2020 American Chemical Society). |
Carbon Dots as Diagnostic Tools or Carriers for Gene Therapy
CDs can be used to fabricate tools to monitor biomarkers for predicting AD risk or monitoring the course of the disease.116–119 This is a rapidly evolving research area, but germane to topics we have previously discussed, CDs or GQDs have been used to develop methods for CSF monitoring of Aβ monomers120 or AChE.121 In addition to acting as a tool to inhibit AchE itself, CDs can also serve as a monitoring and sensing platform based on the acetylcholine hypothesis. Martinez et al122 developed a novel fluorescent biosensor that is based on nitrogen doped GQDs (N-GQDs) as a fluorescent probe and AChE system as a biorecognition element. Among them, N-GQDs were obtained by hydrothermal synthesis using citric acid and ammonia as the carbon and nitrogen sources. The sensor is used to assess tacrine (a cholinesterase inhibitor drug used in the clinical treatment of AD) in aqueous solution. It utilizes the fact that the native fluorescence of N-GQDs is quenched by interaction with enzymatic reaction products, while the fluorescence intensity of N-GQDs is gradually restored in an inhibitor concentration-dependent manner after the addition of tacrine.
Based on the study of N-GQDs, Sharma et al123 took advantage of its high sensitivity and made it a sensing platform to evaluate the in vitro efficacy of novel anti-AD drugs. NGQDs act as fluorescent bioprobes to help reveal the mechanisms associated with AD. The fluorescence alteration of NGQDs helps to understand the effects of anti-AD drugs and how they block AChE activity and inhibit ACh hydrolysis in the brain. NGQDs are less toxic and more biocompatible than nanoparticles,124 CQDs125 or undoped GQDs126 used earlier as sensors or probes. Therefore, their study is the first bold and rational attempt to analyze the potency of newly synthesized derivatives.
MicroRNAs (miRNAs) are conserved tiny non-coding RNAs that affect gene expression at the post-transcriptional level and play a critical role in regulating key genes linked to AD.127 It is worth noting that miR-9, a highly expressed miRNA in neural tissues, is essential for neuronal function and survival. Wu et al46 designed a new type of two-photon fluorescent probe for in situ imaging of miR-9 in living neurons and brain tissues of the transgenic APP/PS1 AD mouse model. The miR-9 probe was composed of graphene oxide, red luminescent carbon dots, and azobenzene-bound DNA (CDs-DNA-Azo). This design combined long-term detection of miR-9 with high sensitivity, selectivity and deep tissue imaging capabilities, while having low cytotoxicity and high biocompatibility. The CDs acted as a two-photon fluorophore, so it was possible to image the probe in deep layers of brain tissue without interference by autofluorescence of the biological matrix (Figure 7A).128 Using both in vivo and in vitro experiments, Wu et al showed that miR-9 in the hippocampus was up-regulated in the early stages of the APP/PS1 model, but was subsequently suppressed to even below the levels seen in wild-type mice at advanced stages of the AD model (Figures 7B and C).
![]() |
Figure 7 (A) Single or two-photon fluorescence imaging of the miR9 probe in hippocampus slices visualized with z-stacking scanning. Scale bar = 100μm. (B) Bar graph showing the relative miR9 probe intensity in the hippocampus of wild-type (control) mice and APP/PS1 mice at 6 and 15 months of age. (C) Two-photon fluorescence images of the hippocampus tissue in normal (wild-type) mice and AD mice at 6 and 15 months old. Boxes define regions of interest (ROIs) used for the quantification of fluorescence intensity shown in (B). Scale bars = 500μm in low-power view of the hippocampus shown at left, and 20μm for remaining images. Excitation wavelength: 960 nm. Used with permission of Royal Society of Chemistry, from Wu W, Zheng T, Tian Y. An enzyme-free amplification strategy based on two-photon fluorescent carbon dots for monitoring miR-9 in live neurons and brain tissues of Alzheimer’s disease mice. Chem Commun. 2020;56(58):8083–8086.; permission conveyed through Copyright Clearance Center, Inc.46 |
Gene therapy has the potential to treat many inherited and acquired human diseases, and the use of nucleic acids (including pDNA, mRNA and non-coding RNA) as gene therapy methods is rapidly entering clinical practice. The key to effective gene therapy is to successfully transport the therapeutic genes to specific targets.129 However, because bare nucleic acid is rapidly destroyed by serum nucleases, an important goal of research to enhance the efficacy of gene therapy is to develop effective vectors that can compress and preserve nucleic acids while also having high gene transfection efficiency130,131 and low toxicity.76 The ability to compress and protect DNA is one of the most important factors of gene delivery vectors.131 Therefore, one of the best strategies for gene delivery is to use CD-compressed pDNA, which can considerably increase gene transfection compared with naked DNA delivery.132
Liu et al37 were among the first to show that CDs can be used as traceable nanocarriers for safe and effective gene delivery. Liu et al successfully constructed a high-efficiency bifunctional nanocarrier (CD-PEI) based on PEI-passivated CDs through microwave-assisted pyrolysis of glycerol in the presence of branched-chain PEI25k. The PEI molecules play two key roles, as surface passivation to endow the CDs with strong photoluminescence, and as a polyelectrolyte to condense the DNA for gene transfection. CD-PEIs shown to have excellent water solubility, to emit stable and bright multicolor fluorescence according to the excitation wavelength, and to have good photostability. The investigators showed that their nanoparticles effectively mediated gene transfection in COS-7 and HepG2 cells. The multicolor fluorescent CD-PEI/DNA complexes was clearly visible in the cytoplasm, demonstrating the potential of CD-PEI in bioimaging and biomarkers. Wang et al133 subsequently extended this work to successfully deliver Survivin siRNA into a human gastric cancer cell line.
In other work, Cao et al134 successfully used CDs to deliver the plasmid SOX9 (pSOX9) to mouse embryonic fibroblasts, and experimentally tested the toxicity of CDs/pSOX9 and its immunogenicity in mice by intravenous injection. These results further demonstrated the biological safety and low toxicity of CDs. However, while evidence continues to accumulate indicating that pathological changes such as amyloid plaques and neurofibrillary tangles seen in AD brain are attributable to specific gene expression disorders,135 to date there are no experimental studies exploring the possibility of combining CDs with genes to treat AD.
Discussion and Future Directions
So far, we have provided evidence that CDs could have a wide number of potential applications for the diagnosis and treatment of AD. However, CDs are still not very mature in terms of drug/gene delivery or bioimaging, and there are still certain limitations that hinder their clinical application. This is an important reason why research is still at the level of in vitro experiments using cell cultures and in vivo experiments in animals. For the clinical application of CDs, the following aspects need to be considered.
Non-toxicity has always been the goal in the treatment of diseases by drugs or nanoparticle (NP) formulations. Some of the formulations previously studied have the potential for toxicity, particularly if they contain lipid moieties. In general, the purity and concentration of NPs will determine their chemical toxicity. For example, in the synthesis of CDs, the main function of N-dopants is to passivate the surface to enhance fluorescence. However, some N-dopants are inherently toxic, but will remain non-toxic at low-medium concentrations. The charge of NPs also affects the toxicity of the particles. Generally speaking, positively charged NPs are usually more toxic than neutral or anionic NPs. In addition, the particle size and shape will also affect its toxicity. For example, only small PLGA NPs can promote inflammation through the release of cytokines. At present, in vivo and clinical data studying the toxic effects of NPs on brain transport are very scarce.
Long-term monitoring has always been an important aspect of experimental research into treatments for AD. However, long-term monitoring requires that NPs have long-term non-toxicity, and preferably features allowing for real-time intracerebral monitoring. Although the non-toxic and autofluorescence properties of CDs meet the experimental needs of real-time monitoring, at present there are no experiments that have been able to achieve experimental monitoring for the many-year period during which AD progresses.
The route of administration can also affect the therapeutic efficacy of CD-carrying drugs. Intracardial and intravenous administration are the methods that have been used to date to assess the capacity for various NP formulations to cross the BBB and reach the brain. Intranasal delivery has attracted attention as an effective delivery method, as drugs can enter the brain directly through the olfactory mucosa, bypassing the BBB. However, due to the reduced dose volume allowed by the nasal cavity, it is theoretically more difficult for drugs delivered through this route to reach therapeutic levels effectively. Finally, oral administration is the most convenient method of drug delivery for patients. Neither intranasal nor oral administration of NPs have been tested to date. Most of the current studies on CDs are still at the in vitro level, and, in particular, there is a paucity in vivo studies of CDs in AD models.
As was mentioned above, research on gene therapy for AD is still at the beginning stages. Gene therapy offers the promise of effective delivery of specific therapeutic genes to unhealthy cells. Compared with only treating specific pathological manifestations, this method could not only relieve pathology but prevent it from developing. Whether the use of CDs can facilitate the development of effective gene delivery therapies for AD remains an open question, and an important one for future research.
In summary, experimental work to date has demonstrated significant potential for the application of CDs in developing new therapeutic interventions for AD. However, in order to realize the application of CDs in the clinical treatment of AD, many issues remain to be investigated or resolved. These include optimizing the size, surface charge, toxicity, biocompatibility of CDs, route of administration, BBB penetration, developing methods for long-term monitoring of delivery and, of course, demonstrating superior efficacy and reduced side effects compared to current treatments. Although they will require substantial additional effort, we are optimistic that these goals can be achieved.
Abbreviations
AD, Alzheimer’s disease; BBB, blood-brain barrier; CDs, carbon dots; Aβ, amyloid β; NFTs, neurofibrillary tangles; NMDA, N-methyl d-aspartate; PL, photoluminescence; CQDs, carbon quantum dots; GQDs, graphene quantum dots; CNDs, carbon nanodots; CPDs, carbonized polymer dots; FA, folic acid; FR, folate receptor; iv, intravenous; im, intramuscular; sc, subcutaneous; EDA, ethylenediamine; BACE1, β-site amyloid precursor protein cleaving enzyme 1; APP, amyloid precursor protein; OPD, o-phenylenediamine; RCDs, red light-absorbing CDs; CUR, Curcumin; ROS, reactive oxygen species; GPE, glycine-proline-glutamate (Gly-Pro-Glu); AChE, acetylcholinesterase; Ach, acetylcholine; Cys, cysteine; miRNAs, MicroRNAs; NP, nanoparticle.
Consent for Publication
The image information quoted in this article has been applied to the relevant publishers and has been approved for use. The graphical abstract was created with BioRender.com.
Funding
This research was funded by the National Natural Science Foundation of China (22071231, 21772189 and 81660224), Fundamental Research Funds for the Central Universities (WK2060000029), Hainan Province Science and Technology Special Fund (Hainan Provincial Natural Science Foundation) of China (821RC562, ZDYF2022SHFZ290, ZDYF2022SHFZ287), Innovative Research Projects for Graduate Students in Hainan Province, China (Qhys2021-353).
Disclosure
The authors declare no conflicts of interest in this work.
References
1. Gaudreault R, Mousseau N. Mitigating Alzheimer’s disease with natural polyphenols: a review. Curr Alzheimer Res. 2019;16(6):529–543. doi:10.2174/1567205016666190315093520
2. Livingston G, Sommerlad A, Orgeta V, et al. Dementia prevention, intervention, and care. Lancet. 2017;390(10113):2673–2734.
3. Nguyen TT, Nguyen TTD, Nguyen TKO, et al. Advances in developing therapeutic strategies for Alzheimer’s disease. Biomed Pharmacother. 2021;139:111623.
4. Lane CA, Hardy J, Schott JM. Alzheimer’s disease. Eur J Neurol. 2018;25(1):59–70.
5. Tiwari S, Atluri V, Kaushik A, et al. Alzheimer’s disease: pathogenesis, diagnostics, and therapeutics. Int J Nanomedicine. 2019;14:5541–5554.
6. Harilal S, Jose J, Parambi DGT, et al. Advancements in nanotherapeutics for Alzheimer’s disease: current perspectives. J Pharm Pharmacol. 2019;71(9):1370–1383.
7. Qian C, Yuan C, Li C, et al. Multifunctional nano-enabled delivery systems in Alzheimer’s disease management. Biomater Sci. 2020;8(20):5538–5554.
8. Niu X, Chen J, Gao J. Nanocarriers as a powerful vehicle to overcome blood-brain barrier in treating neurodegenerative diseases: focus on recent advances. Asian J Pharm Sci. 2019;14(5):480–496.
9. Contestabile A. The history of the cholinergic hypothesis. Behav Brain Res. 2011;221(2):334–340.
10. Parihar MS, Hemnani T. Alzheimer’s disease pathogenesis and therapeutic interventions. J Clin Neurosci. 2004;11(5):456–467. doi:10.1016/j.jocn.2003.12.007
11. Wen MM, El-Salamouni NS, El-Refaie WM, et al. Nanotechnology-based drug delivery systems for Alzheimer’s disease management: technical, industrial, and clinical challenges. J Control Release. 2017;245:95–107. doi:10.1016/j.jconrel.2016.11.025
12. Zorkina Y, Abramova O, Ushakova V, et al. Nano carrier drug delivery systems for the treatment of neuropsychiatric disorders: advantages and limitations. Molecules. 2020;25(22):E5294.
13. Eldufani J, Blaise G. The role of acetylcholinesterase inhibitors such as neostigmine and rivastigmine on chronic pain and cognitive function in aging: a review of recent clinical applications. Alzheimers Dement. 2019;5:175–183.
14. Ramalho MJ, Andrade S, Loureiro JA, et al. Nanotechnology to improve the Alzheimer’s disease therapy with natural compounds. Drug Deliv Transl Res. 2020;10(2):380–402.
15. Daneman R, Prat A. The blood-brain barrier. Cold Spring Harb Perspect Biol. 2015;7(1):a020412.
16. Nisticò R, Borg JJ. Aducanumab for Alzheimer’s disease: a regulatory perspective. Pharmacol Res. 2021;171:105754.
17. Yiannopoulou KG, Papageorgiou SG. Current and future treatments in Alzheimer disease: an update. J Cent Nerv Syst Dis. 2020;12:1179573520907397.
18. Goedert M, Spillantini MG. A century of Alzheimer’s disease. Science. 2006;314(5800):777–781.
19. Duro-Castano A, Moreira Leite D, Forth J, et al. Designing peptide nanoparticles for efficient brain delivery. Adv Drug Deliv Rev. 2020;160:52–77.
20. Sarrazin J-L, Bonneville F, Martin-Blondel G. Brain infections. Diagn Interv Imaging. 2012;93(6):473–490.
21. Burgess A, Hynynen K. Drug delivery across the blood-brain barrier using focused ultrasound. Expert Opin Drug Deliv. 2014;11(5):711–721.
22. Abbott NJ, Rönnbäck L, Hansson E. Astrocyte-endothelial interactions at the blood-brain barrier. Nat Rev Neurosci. 2006;7(1):41–53.
23. Agrahari V. The exciting potential of nanotherapy in brain-tumor targeted drug delivery approaches. Neural Regen Res. 2017;12(2):197–200.
24. Tsou Y-H, Zhang X-Q, Zhu H, et al. Drug delivery to the brain across the blood-brain barrier using nanomaterials. Small. 2017;13:43.
25. Zhou Y, Liyanage PY, Devadoss D, et al. Nontoxic amphiphilic carbon dots as promising drug nanocarriers across the blood-brain barrier and inhibitors of β-amyloid. Nanoscale. 2019;11(46):22387–22397.
26. Zhang W, Sigdel G, Mintz KJ, et al. Carbon dots: a future blood-brain barrier penetrating nanomedicine and drug nanocarrier. Int J Nanomedicine. 2021;16:5003–5016.
27. Li CH, Li RS, Li CM, et al. Precise ricin A-chain delivery by Golgi-targeting carbon dots. Chem Commun. 2019;55(45):6437–6440.
28. Zhou Y, Desserre A, Sharma SK, et al. Gel-like carbon dots: characterization and their potential applications. Chemphyschem. 2017;18(8):890–897.
29. Zheng XT, Ananthanarayanan A, Luo KQ, et al. Glowing graphene quantum dots and carbon dots: properties, syntheses, and biological applications. Small. 2015;11(14):1620–1636.
30. Bao L, Zhang Z-L, Tian Z-Q, et al. Electrochemical tuning of luminescent carbon nanodots: from preparation to luminescence mechanism. Adv Mater. 2011;23(48):5801–5806.
31. Zhu S, Meng Q, Wang L, et al. Highly photoluminescent carbon dots for multicolor patterning, sensors, and bioimaging. Angew Chem Int Ed Engl. 2013;52(14):3953–3957.
32. Jiang K, Zhang L, Lu J, et al. Triple-mode emission of carbon dots: applications for advanced anti-counterfeiting. Angew Chem Int Ed Engl. 2016;55(25):7231–7235.
33. Mansuriya BD, Altintas Z. Carbon dots: classification, properties, synthesis, characterization, and applications in health care-an updated review (2018–2021). Nanomaterials. 2021;11(10):2525.
34. Xia C, Zhu S, Feng T, Yang M, Yang B. Evolution and synthesis of carbon dots: from carbon dots to carbonized polymer dots. Adv Sci. 2019;6:23.
35. Shu Y, Zheng N, Zheng A-Q, et al. Intracellular Zinc quantification by fluorescence imaging with a FRET system. Anal Chem. 2019;91(6):4157–4163.
36. Gao X, Ding C, Zhu A, et al. Carbon-dot-based ratiometric fluorescent probe for imaging and biosensing of superoxide anion in live cells. Anal Chem. 2014;86(14):7071–7078.
37. Liu C, Zhang P, Zhai X, et al. Nano-carrier for gene delivery and bioimaging based on carbon dots with PEI-passivation enhanced fluorescence. Biomaterials. 2012;33(13):3604–3613.
38. Dong H, Dai W, Ju H, et al. Multifunctional Poly(L-lactide)-Polyethylene Glycol-Grafted graphene quantum dots for intracellular MicroRNA imaging and combined specific-gene-targeting agents delivery for improved therapeutics. ACS Appl Mater Interfaces. 2015;7(20):11015–11023.
39. Song Y, Yan X, Li Z, et al. Highly photoluminescent carbon dots derived from linseed and their applications in cellular imaging and sensing. J Mater Chem B. 2018;6(19):3181–3187.
40. Zhang J, Zhao X, Xian M, et al. Folic acid-conjugated green luminescent carbon dots as a nanoprobe for identifying folate receptor-positive cancer cells. Talanta. 2018;183:39–47.
41. Zhou Y, Sharma SK, Peng Z, et al. Polymers in carbon dots: a review. Polymers. 2017;9(2):E67.
42. Bhunia SK, Saha A, Maity AR, et al. Carbon nanoparticle-based fluorescent bioimaging probes. Sci Rep. 2013;3:1473.
43. Zheng M, Ruan S, Liu S, et al. Self-targeting fluorescent carbon dots for diagnosis of brain cancer cells. ACS Nano. 2015;9(11):11455–11461.
44. Li S, Peng Z, Leblanc RM. Method to determine protein concentration in the protein-nanoparticle conjugates aqueous solution using circular dichroism spectroscopy. Anal Chem. 2015;87(13):6455–6459.
45. Peng Z, Li S, Han X, et al. Determination of the composition, encapsulation efficiency and loading capacity in protein drug delivery systems using circular dichroism spectroscopy. Anal Chim Acta. 2016;937:113–118.
46. Wu W, Zheng T, Tian Y. An enzyme-free amplification strategy based on two-photon fluorescent carbon dots for monitoring miR-9 in live neurons and brain tissues of Alzheimer’s disease mice. Chem Commun. 2020;56(58):8083–8086.
47. Ghafary SM, Nikkhah M, Hatamie S, et al. Simultaneous gene delivery and tracking through preparation of photo-luminescent nanoparticles based on graphene quantum dots and chimeric peptides. Sci Rep. 2017;7(1):9552.
48. Xu X, Ray R, Gu Y, et al. Electrophoretic analysis and purification of fluorescent single-walled carbon nanotube fragments. J Am Chem Soc. 2004;126(40):12736–12737.
49. Li H, He X, Kang Z, et al. Water-soluble fluorescent carbon quantum dots and photocatalyst design. Angew Chem Int Ed Engl. 2010;49(26):4430–4434.
50. Kang Z, Lee S-T. Carbon dots: advances in nanocarbon applications. Nanoscale. 2019;11(41):19214–19224.
51. Yang Z-C, Wang M, Yong AM, et al. Intrinsically fluorescent carbon dots with tunable emission derived from hydrothermal treatment of glucose in the presence of monopotassium phosphate. Chem Commun. 2011;47(42):11615–11617.
52. Zhou J, Booker C, Li R, et al. An electrochemical avenue to blue luminescent nanocrystals from multiwalled carbon nanotubes (MWCNTs). J Am Chem Soc. 2007;129(4):744–745.
53. Li S, Wang L, Chusuei CC, et al. Nontoxic carbon dots potently inhibit human insulin fibrillation. Chem Mater. 2015;27(5):1764–1771.
54. Cao L, Wang X, Meziani MJ, et al. Carbon dots for multiphoton bioimaging. J Am Chem Soc. 2007;129(37):11318–11319.
55. Sun Y-P, Zhou B, Lin Y, et al. Quantum-sized carbon dots for bright and colorful photoluminescence. J Am Chem Soc. 2006;128(24):7756–7757.
56. Wang X, Cao L, Lu F, et al. Photoinduced electron transfers with carbon dots. Chem Commun. 2009;25:3774–3776.
57. Kipriianov SM, Petrenko VA. Структурно-функциональная организация белков флакона методом локализованного мутагенеза [Structural-functional organization of vial proteins by a localized mutagenesis method]. Mol Biol. 1991;25(1):5–21. Russian.
58. Zheng L, Chi Y, Dong Y, et al. Electrochemiluminescence of water-soluble carbon nanocrystals released electrochemically from graphite. J Am Chem Soc. 2009;131(13):4564–4565.
59. Liu H, Ye T, Mao C. Fluorescent carbon nanoparticles derived from candle soot. Angew Chem Int Ed Engl. 2007;46(34):6473–6475.
60. Hu S, Trinchi A, Atkin P, et al. Tunable photoluminescence across the entire visible spectrum from carbon dots excited by white light. Angew Chem Int Ed Engl. 2015;54(10):2970–2974.
61. Ðorđević L, Arcudi F, D’Urso A, et al. Design principles of chiral carbon nanodots help convey chirality from molecular to nanoscale level. Nat Commun. 2018;9(1):3442.
62. Li F, Li Y, Yang X, et al. Highly fluorescent chiral N-S-doped carbon dots from cysteine: affecting cellular energy metabolism. Angew Chem Int Ed Engl. 2018;57(9):2377–2382.
63. Arcudi F, Đorđević L, Prato M. Rationally designed carbon nanodots towards pure white-light emission. Angew Chem Int Ed Engl. 2017;56(15):4170–4173.
64. Ding H, Wei J-S, Zhang P, et al. Solvent-controlled synthesis of highly luminescent carbon dots with a wide color gamut and narrowed emission peak widths. Small. 2018;14(22):e1800612.
65. Krysmann MJ, Kelarakis A, Dallas P, et al. Formation mechanism of carbogenic nanoparticles with dual photoluminescence emission. J Am Chem Soc. 2012;134(2):747–750.
66. Wu F, Su H, Wang K, et al. Facile synthesis of N-rich carbon quantum dots from porphyrins as efficient probes for bioimaging and biosensing in living cells. Int J Nanomedicine. 2017;12:7375–7391.
67. Yao H, Li J, Song Y, et al. Synthesis of ginsenoside Re-based carbon dots applied for bioimaging and effective inhibition of cancer cells. Int J Nanomedicine. 2018;13:6249–6264.
68. Hu Y, Yang J, Tian J, et al. How do nitrogen-doped carbon dots generate from molecular precursors? An investigation of the formation mechanism and a solution-based large-scale synthesis. J Mater Chem B. 2015;3(27):5608–5614.
69. Xu H, Yan L, Nguyen V, et al. One-step synthesis of nitrogen-doped carbon nanodots for ratiometric pH sensing by femtosecond laser ablation method. Appl Surf Sci. 2017;414:238–243.
70. Zhai X, Zhang P, Liu C, et al. Highly luminescent carbon nanodots by microwave-assisted pyrolysis. Chem Commun. 2012;48(64):7955–7957.
71. Suner SS, Sahiner M, Ayyala RS, et al. Versatile fluorescent carbon dots from citric acid and cysteine with antimicrobial, anti-biofilm, antioxidant, and AChE enzyme inhibition capabilities. J Fluoresc. 2021;31(6):1705–1717.
72. Zhang J, Yuan Y, Liang G, et al. Scale-up synthesis of fragrant nitrogen-doped carbon dots from bee pollens for bioimaging and catalysis. Adv Sci. 2015;2(4):1500002.
73. Miao X, Qu D, Yang D, et al. Synthesis of carbon dots with multiple color emission by controlled graphitization and surface functionalization. Adv Mater. 2018;30(1):1704740.
74. Ge J, Jia Q, Liu W, et al. Red-emissive carbon dots for fluorescent, photoacoustic, and thermal theranostics in living mice. Adv Mater. 2015;27(28):4169–4177.
75. Chong Y, Ma Y, Shen H, et al. The in vitro and in vivo toxicity of graphene quantum dots. Biomaterials. 2014;35(19):5041–5048.
76. Mohammadinejad R, Dadashzadeh A, Moghassemi S, et al. Shedding light on gene therapy: carbon dots for the minimally invasive image-guided delivery of plasmids and noncoding RNAs – A review. J Adv Res. 2019;18:81–93.
77. Yang S-T, Cao L, Luo PG, et al. Carbon dots for optical imaging in vivo. J Am Chem Soc. 2009;131(32):11308–11309.
78. Choi HS, Liu W, Misra P, et al. Renal clearance of quantum dots. Nat Biotechnol. 2007;25(10):1165–1170.
79. Huang X, Zhang F, Zhu L, et al. Effect of injection routes on the biodistribution, clearance, and tumor uptake of carbon dots. ACS Nano. 2013;7(7):5684–5693.
80. Bäckman C, Rose GM, Hoffer BJ, et al. Systemic administration of a nerve growth factor conjugate reverses age-related cognitive dysfunction and prevents cholinergic neuron atrophy. J Neurosci. 1996;16(17):5437–5442.
81. Johnsen KB, Burkhart A, Melander F, et al. Targeting transferrin receptors at the blood-brain barrier improves the uptake of immunoliposomes and subsequent cargo transport into the brain parenchyma. Sci Rep. 2017;7(1):10396.
82. Li S, Amat D, Peng Z, et al. Transferrin conjugated nontoxic carbon dots for doxorubicin delivery to target pediatric brain tumor cells. Nanoscale. 2016;8(37):16662–16669.
83. Li S, Peng Z, Dallman J, et al. Crossing the blood-brain-barrier with transferrin conjugated carbon dots: a zebrafish model study. Colloids Surf B Biointerfaces. 2016;145:251–256.
84. Mintz KJ, Mercado G, Zhou Y, et al. Tryptophan carbon dots and their ability to cross the blood-brain barrier. Colloids Surf B Biointerfaces. 2019;176:488–493.
85. Seven ES, Seven YB, Zhou Y, et al. Crossing the blood-brain barrier with carbon dots: uptake mechanism and in vivo cargo delivery. Nanoscale Adv. 2021;3(13):3942–3953.
86. Han X, Jing Z, Wu W, et al. Biocompatible and blood-brain barrier permeable carbon dots for inhibition of Aβ fibrillation and toxicity, and BACE1 activity. Nanoscale. 2017;9(35):12862–12866.
87. Hung A, Griffin MDW, Howlett GJ, et al. Effects of oxidation, pH and lipids on amyloidogenic peptide structure: implications for fibril formation? Eur Biophys J. 2008;38(1):99–110.
88. Todorova N, Makarucha AJ, Hine NDM, et al. Dimensionality of carbon nanomaterials determines the binding and dynamics of amyloidogenic peptides: multiscale theoretical simulations. PLoS Comput Biol. 2013;9(12):e1003360.
89. Yan C, Wang C, Shao X, et al. Multifunctional carbon-dot-photosensitizer nanoassemblies for inhibiting amyloid aggregates, suppressing microbial infection, and overcoming the blood-brain barrier. ACS Appl Mater Interfaces. 2022. doi:10.1021/acsami.2c14118
90. Curtain CC, Ali F, Volitakis I, et al. Alzheimer’s disease amyloid-beta binds copper and zinc to generate an allosterically ordered membrane-penetrating structure containing superoxide dismutase-like subunits. J Biol Chem. 2001;276(23):20466–20473.
91. Lovell MA, Robertson JD, Teesdale WJ, et al. Copper, iron and zinc in Alzheimer’s disease senile plaques. J Neurol Sci. 1998;158(1):47–52.
92. Benilova I, Karran E, De Strooper B. The toxic Aβ oligomer and Alzheimer’s disease: an emperor in need of clothes. Nat Neurosci. 2012;15(3):349–357.
93. Syme CD, Nadal RC, Rigby SEJ, et al. Copper binding to the amyloid-beta (Abeta) peptide associated with Alzheimer’s disease: folding, coordination geometry, pH dependence, stoichiometry, and affinity of Abeta-(1–28): insights from a range of complementary spectroscopic techniques. J Biol Chem. 2004;279(18):18169–18177.
94. Chung YJ, Lee BI, Park CB. Multifunctional carbon dots as a therapeutic nanoagent for modulating Cu(ii)-mediated β-amyloid aggregation. Nanoscale. 2019;11(13):6297–6306.
95. Chung YJ, Lee CH, Lim J, et al. Photomodulating carbon dots for spatiotemporal suppression of Alzheimer’s β-amyloid aggregation. ACS Nano. 2020;14(12):16973–16983.
96. Ak T, Gülçin I. Antioxidant and radical scavenging properties of curcumin. Chem Biol Interact. 2008;174(1):27–37.
97. Esatbeyoglu T, Huebbe P, Ernst IMA, et al. Curcumin–from molecule to biological function. Angew Chem Int Ed Engl. 2012;51(22):5308–5332.
98. Shabbir U, Rubab M, Tyagi A, et al. Curcumin and its derivatives as theranostic agents in Alzheimer’s disease: the implication of nanotechnology. Int J Mol Sci. 2020;22(1):E196.
99. Kuang Y, Zhang J, Xiong M, et al. A novel nanosystem realizing curcumin delivery based on [email protected] dots nanocomposite for Alzheimer’s disease therapy. Front Bioeng Biotechnol. 2020;8:614906.
100. Zheng K, Dai X, Xiao N, et al. Curcumin ameliorates memory decline via inhibiting BACE1 Expression and β-Amyloid pathology in 5×FAD transgenic mice. Mol Neurobiol. 2017;54(3):1967–1977. doi:10.1007/s12035-016-9802-9
101. Perini G, Palmieri V, Ciasca G, et al. Enhanced chemotherapy for glioblastoma multiforme mediated by functionalized graphene quantum dots. Materials. 2020;13(18):E4139. doi:10.3390/ma13184139
102. Liu Y, Xu L-P, Dai W, et al. Graphene quantum dots for the inhibition of β amyloid aggregation. Nanoscale. 2015;7(45):19060–19065. doi:10.1039/C5NR06282A
103. Xiao S, Zhou D, Luan P, et al. Graphene quantum dots conjugated neuroprotective peptide improve learning and memory capability. Biomaterials. 2016;106:98–110. doi:10.1016/j.biomaterials.2016.08.021
104. Liu Y, Xu L-P, Wang Q, et al. Synergistic inhibitory effect of GQDs–tramiprosate covalent binding on amyloid aggregation. ACS Chem Neurosci. 2018;9(4):817–823. doi:10.1021/acschemneuro.7b00439
105. Manzano S, Agüera L, Aguilar M, et al. A review on tramiprosate (Homotaurine) in Alzheimer’s disease and other neurocognitive disorders. Front Neurol. 2020;11:614. doi:10.3389/fneur.2020.00614
106. Li H, Zhang Y, Ding J, et al. Synthesis of carbon quantum dots for application of alleviating amyloid-β mediated neurotoxicity. Colloids Surf B Biointerfaces. 2022;212:112373. doi:10.1016/j.colsurfb.2022.112373
107. Swerdlow RH, Burns JM, Khan SM. The Alzheimer’s disease mitochondrial cascade hypothesis: progress and perspectives. Biochim Biophys Acta. 2014;1842(8):1219–1231. doi:10.1016/j.bbadis.2013.09.010
108. Holtzman DM, Morris JC, Goate AM. Alzheimer’s disease: the challenge of the second century. Sci Transl Med. 2011;3(77):77sr1. doi:10.1126/scitranslmed.3002369
109. Johnson KA, Schultz A, Betensky RA, et al. Tau positron emission tomographic imaging in aging and early Alzheimer disease. Ann Neurol. 2016;79(1):110–119. doi:10.1002/ana.24546
110. Zhang W, Arteaga J, Cashion DK, et al. A highly selective and specific PET tracer for imaging of tau pathologies. J Alzheimers Dis. 2012;31(3):601–612. doi:10.3233/JAD-2012-120712
111. Zhang W, Kandel N, Zhou Y, et al. Drug delivery of memantine with carbon dots for Alzheimer’s disease: blood–brain barrier penetration and inhibition of tau aggregation. J Colloid Interface Sci. 2022;617:20–31. doi:10.1016/j.jcis.2022.02.124
112. Zhou Y, Kandel N, Bartoli M, et al. Structure-activity relationship of carbon nitride dots in inhibiting tau aggregation. Carbon N Y. 2022;193:1–16. doi:10.1016/j.carbon.2022.03.021
113. Liyanage PY, Zhou Y, Al-Youbi AO, et al. Pediatric glioblastoma target-specific efficient delivery of gemcitabine across the blood–brain barrier via carbon nitride dots. Nanoscale. 2020;12(14):7927–7938. doi:10.1039/D0NR01647K
114. Taranalli AD, Cheeramkuzhy TC. Influence of clitoria ternatea extracts on memory and central cholinergic activity in rats. Pharm Biol. 2000;38(1):51–56. doi:10.1076/1388-0209(200001)3811-BFT051
115. Tak K, Sharma R, Dave V, et al. Clitoria ternatea mediated synthesis of graphene quantum dots for the treatment of Alzheimer’s disease. ACS Chem Neurosci. 2020;11(22):3741–3748. doi:10.1021/acschemneuro.0c00273
116. Carneiro P, Morais S, Pereira MC. Nanomaterials towards biosensing of Alzheimer’s disease biomarkers. Nanomaterials. 2019;9(12):E1663. doi:10.3390/nano9121663
117. Toyos-Rodríguez C, García-Alonso FJ, de la Escosura-Muñiz A. Electrochemical biosensors based on nanomaterials for early detection of Alzheimer’s disease. Sensors. 2020;20(17):E4748. doi:10.3390/s20174748
118. Gopalan D, Pandey A, Alex AT, et al. Nanoconstructs as a versatile tool for detection and diagnosis of Alzheimer biomarkers. Nanotechnology. 2021;32(14):142002.
119. Guo Y, Liu D, Yang Q, et al. Early and in-stage diagnosis of Alzheimer’s disease by the simultaneous fluorescent determination of the biomarkers miR-501-3p and miR-455-3p with carbon quantum dots in serum. Anal Lett. 2022;55(9):1453–1465.
120. Liu C, Lu D, You X, et al. Carbon dots sensitized lanthanide infinite coordination polymer nanoparticles: towards ratiometric fluorescent sensing of cerebrospinal Aβ monomer as a biomarker for Alzheimer’s disease. Anal Chim Acta. 2020;1105:147–154.
121. Liu C, Huang C, Ma R, et al. Cu2+-Regulated reversible coordination interaction of [email protected]/GMP ICP nanoparticles: towards directly monitoring cerebrospinal acetylcholinesterase as a biomarker for cholinic brain dysfunction. Analyst. 2021;145(24):7849–7857.
122. Benítez-Martínez S, Caballero-Díaz E, Valcárcel M. Development of a biosensing system for tacrine based on nitrogen-doped graphene quantum dots and acetylcholinesterase. Analyst. 2016;141(9):2688–2695.
123. Sharma S, Singh N, Nepovimova E, et al. Interaction of synthesized nitrogen enriched graphene quantum dots with novel anti-Alzheimer’s drugs: spectroscopic insights. J Biomol Struct Dyn. 2020;38(6):1822–1837.
124. Zhu W, Saddam Khan M, Cao W, et al. Ni(OH)2/NGQDs-based electrochemiluminescence immunosensor for prostate specific antigen detection by coupling resonance energy transfer with [email protected] composites. Biosens Bioelectron. 2018;99:346–352.
125. Ensafi AA, Nasr-Esfahani P, Rezaei B. Synthesis of molecularly imprinted polymer on carbon quantum dots as an optical sensor for selective fluorescent determination of promethazine hydrochloride. Sensors Actuators B. 2018;257:889–896.
126. Liu Z-T, Ye J-S, Hsu S-Y, et al. A sonoelectrochemical preparation of graphene nanosheets with graphene quantum dots for their use as a hydrogen peroxide sensor. Electrochim Acta. 2018;261:530–536.
127. Delay C, Mandemakers W, Hébert SS. MicroRNAs in Alzheimer’s disease. Neurobiol Dis. 2012;46(2):285–290.
128. Sun S, Zhang L, Jiang K, et al. Toward high-efficient red emissive carbon dots: facile preparation, unique properties, and applications as multifunctional theranostic agents. Chem Mater. 2016;28(23):8659–8668.
129. Dunbar CE, High KA, Joung JK, et al. Gene therapy comes of age. Science. 2018;359(6372):eaan4672.
130. Niven R, Pearlman R, Wedeking T, et al. Biodistribution of radiolabeled lipid-DNA complexes and DNA in mice. J Pharm Sci. 1998;87(11):1292–1299.
131. He X, Chen P, Zhang J, et al. Cationic polymer-derived carbon dots for enhanced gene delivery and cell imaging. Biomater Sci. 2019;7(5):1940–1948.
132. Dou Q, Fang X, Jiang S, et al. Multi-functional fluorescent carbon dots with antibacterial and gene delivery properties. RSC Adv. 2015;5(58):46817–46822.
133. Wang Q, Zhang C, Shen G, et al. Fluorescent carbon dots as an efficient siRNA nanocarrier for its interference therapy in gastric cancer cells. J Nanobiotechnol. 2014;12:58.
134. Cao X, Wang J, Deng W, et al. Photoluminescent cationic carbon dots as efficient non-viral delivery of plasmid SOX9 and chondrogenesis of fibroblasts. Sci Rep. 2018;8(1):7057.
135. Sims R, Hill M, Williams J. The multiplex model of the genetics of Alzheimer’s disease. Nat Neurosci. 2020;23(3):311–322.