Mussel-Based Biomimetic Strategies in Musculoskeletal Disorder Treatment: From Synthesis Principles to Diverse Applications
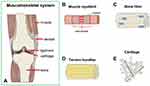
Introduction
Mussels are a common marine organism, of which mussel foot protein (Mfp) is the most representative natural adhesion substance with excellent adhesion, great flexibility, superior biocompatibility, and low toxicity, which has attracted increasing attention. The development of promising mussel biomimetic adhesion materials by mimicking the molecular structure and properties of natural mussel adhesion proteins has become a research hotspot in a spectrum of research fields such as mussel biomimetic,1 biomedical engineering,2–4 soft robotics, electronics,5–7 environmental science,8–10 energy science,11,12 and so forth. In particular, a library of mussel-inspired materials, mainly including polydopamine (PDA), PDA-coated materials, and catechol-based polymers,13–16 have been exploited as essential building blocks to improve musculoskeletal system regeneration.
The musculoskeletal system offers mechanical support to the human body, consisting of hard (bone) and soft tissues (cartilage, muscles, tendons, and ligaments),17 as shown in Figure 1. When injuries and disorders (especially in the musculoskeletal system) affect the human body’s movement, such as osteochondral defects, osteosarcoma, osteoarthritis, ligament rupture, and osteoporosis, it is termed as musculoskeletal disorders (MSDs). MSDs are the second leading cause of disability worldwide, which has posed a huge global burden to public sanitation systems.18,19 Surgical intervention involving device/biomaterial implantation is currently indispensable to treat MSDs but frequently displayed a short-term and temporary therapeutic effect.20 In recent years, approaches in regenerative tissue engineering have been widely employed to treat interface injuries or fill defects (scaffolds),21–23 exhibiting promising potential over other strategies that simply apply inert materials to assist the therapy. As one of the critical factors in regenerative tissue engineering, the selection and utilization of biomimetic materials will play a significant role. Over the last two decades, outstanding success has been achieved in mussel-inspired biomaterials, mainly from the design and synthesis of diverse mussel-inspired compounds to the exploitation of potential applications in the field of MSDs. However, hitherto, there have been only two review articles on mussel-inspired biomaterials with a sparse focus on the applications in MSDs,24,25 to which the tremendous vigor in this emerging field displays striking contrast. Therefore, it is important to shed light on the recent progress and future trend in this field.
![]() |
Figure 1 (A) The schematic illustration shows the components of the musculoskeletal system. (B) Muscle myofibril is composed of endomysium, perimysium, and epimysium. Cell types: Myocytes. (C) Bone fiber is composed of trabecular and cortical bone. Cell types: Osteoclast; osteoblast; osteocyte (D) Tendon bundles are composed of endotenon, epitenon, and peritendon. Cell types: Tenocytes (E) Cartilage is composed of the superficial, middle, and deep zone. Cell types: Chondrocytes. Reproduced with permission from: Casanellas I, et al. Producing 3D Biomimetic Nanomaterials for Musculoskeletal System Regeneration. Front Bioeng Biotechnol. 2018;6:128. doi: 10.3389/fbioe.2018.00128.126 Copyright 2018. Casanellas, García-Lizarribar, Lagunas and Samitier. |
Herein, we initially introduce the main categories and characteristics of Mfps, as well as the fundamental mechanisms underpinning the spectacular adhesion in mussels. Subsequently, we elaborate on the diverse synthetic methods and modifications of various polymers. Moreover, the cutting-edge applications in MSDs are then highlighted, including treatments of osteochondral defects, osteosarcoma, osteoarthritis, ligament rupture, and osteoporosis. Additionally, the remaining challenges and future perspectives are discussed in depth, aiming to provide a unique and insightful perspective to improve the development of innovative mussel biomimetic strategies.
Mussels: From Macro to Micro Scales
Morphology and Formation Process of Mussel Byssus: The Macro Scale
The key for mussel’s survival in coastal habitats is its byssal attachment which offers strong adhesive properties on diverse surfaces. Byssus, a high-performance fibrous material, is produced by mussels to withstand waves and protect themselves against predators.26 Currently, byssus-mediated adhesion between clustered and individual mussels is thoroughly studied and widely applied to marine fixtures. Mussel foot exhibits vigorous synthetic activity to produce a thread in their ventral groove at once to form a complete byssus (Figure 2A).27 Notably, due to the mussels’ age difference, the byssus production rates range from 30 sec to 8 min (young mussels are relatively faster).28 The thread formation is similar to liquid transfer in microfluidic devices: three main gland reservoirs, phenol, collagen, and accessory glands, quantitatively fill their contents into the ventral groove (Figure 2A).29,30 These glands are responsible for synthesizing and storing molecular components that adhere to plaques, collagen cores, and the cuticle. Secreted proteins produced by these glands are injected from the top to the bottom of the ventral groove to form the initial byssus. The ventral groove on the mussel foot is the mold for the byssus generation, while its distal depression is the location for plaque formation (Figure 2A).
![]() |
Figure 2 (A) Schematic illustration shows the holistic appearance of the mussel and the structure of the mussel’s foot and substratum. The formation of byssus is similar to the reminiscent of reaction injection molding, which is explained in the text in detail. (B) The schematic illustration shows the location of different types of Mfps and other known proteins in the plaque and distal thread. Adapted with permission from: Waite, J.H. Mussel adhesion – essential footwork. J Exp Biol. 2017;220(Pt 4):517–530. doi: 10.1242/jeb.134056.32 Copyright 2017. The Company of Biologists Ltd. |
Finally, just before the thread departs from the groove, the assembled structure is coated with an approximately 5-µm-thick layer of the cuticle from accessory glands, so the new thread is used for load bearing purpose. Although the characteristics of byssus have not been completely elaborated, there are more than 20 known protein components, most of which display a highly localized distribution (Figure 2B). Mussel foot proteins, especially Mfp2, 3, 4, and 5, originate from the phenol gland, and distribute mainly in the plaque, playing a significant role in its adhesive functions.
The Representative Categories of Mfps: The Micro Scale
Mfps are divided into six subcategories according to their distribution in the foot,28,31–33 as shown in 2B and Table 1, of which Mfp3 and Mfp5 are the most studied. They are located at the bottom of the foot plaque, acting as the main proteins for intense bio-adhesion.34,35 Mfp3 is the smallest but most polymorphic Mfp identified thus far, with 30–35 variants, which are classified into fast-type Mfp3f and slow-type Mfp3s based on the electrophoresis rate. Mfp5 contains positively charged amino acid residues (approximately 20 mol% positive residues) and a phosphoserine residue with an extra-frontal negative charge (approximately 10 mol% negative residues). Other Mfps also play a significant role in mussel foot filament morphology maintenance and mussel adhesion. Mfp1 is composed of 80 tandem repeat decapeptides, which mainly protect the foot silk core and foot silk plaque against certain hard substances such as rocks. Mfp4 mainly mediates the morphological transformation of the byssus from the thread to the plaque.36,37 Mfp2 is located among various Mfps (Mfp4, Mfp3, Mfp5, and Mfp6), serving as the most abundant adhesion protein in the plaque, accounting for approximately 25% of the total proteins. The Mfp2 contains 6~7 mol% Cys residues, and includes 11 tandem epidermal growth factor-like motifs, which are intramolecularly connected by the disulfide bonds, suggesting a high stability improvement in the byssus.36,38,39 Mfp6 locate at the bottom of the mussel foot plaque together with Mfp3 and Mfp5 in which Cys content is even higher (11 mol%). It can form a Cys-DOPA cross-linking bond with Mfp3 and Mfp5 to avoid 3,4-dihydroxyphenylalanine (DOPA) oxidation, and the sulfhydryl group in Cys is employed to reduce dopaquinone to DOPA to enhance the adhesion of Mfp3 and Mfp5 to the substrate.36
![]() |
Table 1 Biochemical Comparison of the Mfps in the Adhesive Plaques and Threads Regarding Mass, pI, DOPA Content, Localization of Proteins in the Mussel Byssus, Sequence, and Metal Binding |
The Mechanism Behind the Mystery of Mussel Adhesion
Mfp is able to anchor to various organic or inorganic surfaces without quick tearing, mainly due to its intense adhesion and cohesion.40–42 In the adhesion process, DOPA in Mfp can attach to different substrates through various interactions, including hydrogen bond, metal-catechol coordination bond, π‐π/π‐ cation interaction, and others with the schematic illustrations and details shown in Table 2.39 Taking the interaction between DOPA and TiO2 surface as an example, catechol on the DOPA side chain can form hydrogen bonds with the TiO2 surface, and the connection between catechol and O2- is transformed from a bidentate hydrogen bond to a bidentate coordination bond with Ti4+ accompanied by pH increase.43 In terms of cohesion, the attraction between neighboring parts is a manifestation of the molecular forces. For instance, the interaction between DOPA in Mfp and cation ‐π in Lys greatly promotes Mfp cohesion so that it lays a solid foundation for the superior adhesion of mussels.36 In addition, other aromatic amino acid residues (eg, Phe and Tyr residues) also contribute to the intense cation ‐π interactions by providing cohesion. Based on the adhesion mechanisms discussed above, it is expected that diverse innovative materials and devices would be proposed by mimicking the Mfp structure and properties.
Mussel Biomimetic Materials: From Structure Design to Synthetic Optimization
Currently, there are mainly three ways to obtain mussel-biomimetic adhesive materials: first, extract and isolate adhesive proteins directly from marine mussel byssus, which is the most convenient, straightforward, and effective approach.44 However, it is unsuitable for large-scale sample preparation due to the limited mussel size, minimal adhesive protein secretion, the complex extraction process, extremely low yield, and high cost. Second, gene engineering is utilized to recombine relevant genes into Escherichia coli or yeast for specific protein expression.45–47 Compared with the first method, the yield of adhesive protein is remarkably increased, but the adhesion performance fails to meet the standard of natural mussels.33 Third, a synthetic polymer with a catechol adhesive group is used to mimic the adhesion property of marine mussels by incorporating DOPA into the side chain or the end of the polymer skeleton.48 It is the most studied and widely used strategy to create mussel-biomimetic adhesive materials. According to the diverse polymer skeletons, it is typically divided into three subtypes that are described as follows.
Catechol-Adhesion Polypeptide Copolymer
Mfp5 is one of the most important proteins in the byssal adhesive plaque of the mussel,49 which mainly consists of glycine, L-lysine, and DOPA.50 The high ratio of DOPA (∼30 mol %) and its specific distribution (near the plaque−substrate interface) indicate its significance in the bio-adhesion.51 By mimicking the composition of Mfp5, mussel-biomimetic adhesive copolymers (polypeptide) are synthesized via chemoenzymatic, solution-based, ring-opening polymerization, introducing catechol group into the side chain of the polypeptide backbone. For instance, in chemoenzymatic approach, L-tyrosine and L-lysine residues are initially linked into polypeptides, and L-tyrosine is subsequently converted into DOPA catalyzed by tyrosinase, which enhances the polymerization degree of the copolymer and increases the yield, thus improving the synthesis efficiency of the peptide-based materials. Notably, as a scaffold surface-coating material, these materials are broadly applied in the medical field.52–54 From the end of the twentieth century, the solution-based synthesis method was invented to prepare DOPA-containing poly amino acids,55,56 including various PDA and polypeptide polymers. The synthetic polymers are water-soluble, cross-linked by tyrosinase, presenting high adhesion properties similar to mussels. Moreover, Wang et al57 synthesized DOPA- and lysine-containing polypeptides by ring-opening polymerization in which ring monomers are connected to each other to form linear polymers, and introduced iron ions into the system to optimize the bond strength and water resistance, suggesting high functional tunability.
Catechol – Polysaccharide Polymer
Polysaccharide is a kind of natural polymer consisting of aldose or ketose connected by glycosidic bonds, and widely exists in natural animals and plants, which is safe, non-toxic, easy to extract and water-soluble.58 The -OH and NH2 functional groups in the polysaccharide act as the active center to dominate the crosslinking reaction. The catechol was grafted to those groups to generate a cross-linked three-dimensional network, facilitating large-scale biomaterial formation and application.59
Self-Polymerization of Dopamine and Its Derivatives
PDA is mainly extracted from mussel adhesion protein and displays strong wet adhesion to the matrix.60 It can be traced back to 2007 when Messersmith et al found that the self-polymerization of dopamine was able to create a thin and surface-adherent PDA film on the surface of various inorganic and organic materials.42 Since then, many other researchers have followed this strategy to modify polysaccharides in various fields including tissue engineering and wound healing, to name only a few, which endows the polysaccharides with diverse promising functions, such as excellent adhesion, oxidation resistance, antibacterial ability, high reactivity, chelation, corrosion resistance, biocompatibility, and so forth.61,62
Grafting Dopamine to Polysaccharides
In addition to self-polymerization, a myriad of polysaccharides enable dopamine graft through covalent bonds based on existing functional groups, including -NH2, -COOH, -OH, and -SH. These groups were grafted with catechol to generate a three-dimensional cross-linked network. Notably, there are mainly three methods to graft specific groups to prepare catechol polysaccharides, including chemical, electrochemical, and enzymatic methods, among which electrochemical methods are the most commonly applied.63 Taking chitosan as an example, the methods of integrating catechol to the main chain of chitosan are classified according to the following three strategies: (1) Using 1-(3-dimethylaminopropyl)-3-ethyl carbodiimide hydrochloride (EDC) and N-hydroxy succinimide (NHS) to chemically build amide bonds. Hu et al64 employed quaternary ammonium chitosan grafted with tyrosine as raw material, which was grafted with EDC and NHS, and then introduced tyrosine to enhance the mechanical properties. This strategy endows chitosan with high adaptation to complicated vascular structures, indicating great potential as a promising scaffold material. (2) Direct oxidative coupling of catechol to amino groups in chitosan. Zhang et al65 applied NaIO4 to oxidize catechol to quinone and reduced the byproduct production via the Michael addition reaction or Schiff base reaction accompanied by the cross-linking agent decrease. (3) Incorporating the amino group with an aldehyde group at one end to produce chitosan-catechol. It is arduous to chemically link the primary amine with polyethylene glycol catechol as the classic reaction between the primary amine and carboxyl group is main to form amide bonds. To overcome this challenge, Hong et al66 chose to produce secondary amines through aldehyde chemistry, which is termed as reductive amination reaction.
Catechol-Adhesion Protein Polymer
The coupling location of polymers such as polyethylene glycol, polyethyleneimine, polyacrylamide (PAM), and polystyrene are mainly in the side chain or the end of the skeleton, which are linked to catechol-contained polymers to create new materials with superior stability, selectivity, and reactivity. For example, Or Berger et al67 introduced peptide units as side chains on brush polymer, rather than being linearly arrayed in natural proteins. Intriguingly, it demonstrated obviously high adhesion after modification even over natural proteins. Lu et al13,68 prepared a mussel-like, super stretched, self-healing hydrogel that held a two-dimensional space of nano clay inside. DOPA was intercalated into the clay to facilitate PDA production during polymerization, which was oxidized in a narrow space that would reduce the oxidation rate, thus maintaining long-term adhesion performance. Subsequently, acrylamide, initiator, and cross-linking agents were added to PDA clay, which remarkably enhance the toughness and tensile properties of the hydrogel, improving the following adhesion and drug delivery.
The Applications of Mussel Biomimetic Materials in MSDs
Induced by injuries, tumors, and many other pathological factors, MSDs remain a great challenge in clinical practice. Nowadays, the application of biomaterials especially adhesives has provided a potential strategy for MSDs as a result of its biocompatibility, degradability, and particularly strong adhesion ability.69 However, the biofunction of regular materials such as the invasive inert materials that only serve as a support for broken bones is usually limited, which cannot meet demands in specific application scenarios, such as immunomodulation and antibiosis, to name only a few. Moreover, the direct extraction of relatively pure adhesives from living organisms is also a challenge. Thus, this section mainly focuses on physical/chemical modification of mussel-based biomimetic materials and their applications in MSDs as shown in Figure 3, including osteochondral defects, osteosarcoma, osteoarthritis, ligament rupture, and osteoporosis. The research stages and treatment outcomes of these biomimetic mussel materials are included in Table 3.
![]() |
Table 3 Diverse Applications of Mussel Biomimetic Materials Used in MSDs Along with Research Stages and Outcomes |
![]() |
Figure 3 Schematic illustration of bioinspired adhesive formulations based on biomimetic strategies. (Created with BioRender.com). |
Osteochondral Defect Treatment
Osteochondral defects, involving the fracture of both articular cartilage and subchondral bone, are a tremendous challenge to repair due to the complex hierarchical architecture of the osteochondral tissue.70 Nevertheless, it is proved that mussel-based biomimetic materials could play a significant role as surface coatings, adhesives, hydrogels, etc., in osteochondral regeneration.71–73 Gan et al74 intercalated ODMA into GelMA hydrogel to enhance toughness and resilience, which was verified to serve as a growth-factor-free support for cartilage regeneration. Given that the applied materials slack specific flexibility to improve adaptation to the human body environment, subtle advancements are needed to improve shape alteration and duration. More encouragingly, Han et al75 designed a mussel-inspired PDA-CS-PAM hydrogel through PDA integrating strategy, which enhanced the tissue repairing potential of various materials even that were cell-repellent. The PDA and CS self-assembled into a cartilage-specific PDA−CS complex, which was incorporated into the hydrogel and then covalently cross-linked to the PAM network, imparting the hydrogel with high toughness and resilience. In addition, the photo-crosslinked hydrogel scaffold designed by Ju et al76 can accelerate cartilage regeneration via recruiting endogenous TGF-β1 and inducing the differentiation of mesenchymal stem cells into chondrocytes, which provided a potential system for clinical cartilage tissue repair.
Compared with homogenized single-layer hydrogels that only partially restore the osteochondral tissue’s original function, researchers from Lu’s team77 developed a mussel-inspired bilayer hydrogel that was efficient in osteochondral defect repair. A one-pot method was ingeniously employed to simultaneously generate upper and lower layers.78 The upper layer acted as a cartilage repair layer, while the lower one served as a subchondral bone regeneration layer. Notably, traditional 2D or 3D structures were rarely responsible for osteochondral microenvironment reconstruction, thus scientists have already invented a 4D membrane for to enhance bone repair via both structure and microenvironment adjustment. Based on the adhesion of mussels, Liu et al79 optimized the microstructure of PDA-anchored grafts, and successfully prepared porous elastomer scaffolds by mixing the thermoplastic PGS with an appropriate amount of PCL to obtain 3D printed additives with desirable mechanical strength and degradation rate (Figure 4). Additionally, the immunomodulatory effect of PDA coatings is significantly enhanced by the proper design of channels and intrinsic pores in the microstructures. This 4D-PDA membrane, taking PDA as an ideal polymer membrane surface modifier, has been widely used to improve the interfacial environment between the implant and host owing to its excellent biocompatibility, mild synthesis conditions, and effective drug delivery capability.72
![]() |
Figure 4 4D-PDA membrane enhances bone regeneration by recruiting BMSCs and accelerating osteogenesis. (A) The schematic diagram illustrates that M2 macrophages mediated by the elastic membrane of 4D stratified channel are enriched in the early and persistent period above the bone defect, which contributes to the formation of bone regeneration with specific shape. (B) Schematic illustration of 4D membrane fabrication process. (C) Schematic illustration of the application process of 4D-PDA membrane in rat calvarial bone defects. (D) H&E staining of the regenerated bone and the quantitative analysis at 4 and 12 weeks after implantation. (E) Immunohistochemistry staining of CD146, STRO-1, Runx2, and BMP2 in the defect areas and the semi-quantification of positive cells in the staining at 4 and 12 weeks. Reprinted from Biomaterials, Volume 276, Liu X, Chen W, Shao B, et al, Mussel patterned with 4D biodegrading elastomer durably recruits regenerative macrophages to promote regeneration of craniofacial bone, Pages No. 120998, Copyright (2021), with permission from Elsevier.79 #p>0.05, *p<0.05 and **p<0.01. |
In recent years, mussel-based biomimetic strategies have been applied to treat periodontal bone defects caused by periodontitis, occlusal trauma, and congenital malformations.80–82 Diverse biomaterials, including nanoparticles83,84 and nanohydrogels,85 have been developed to treat periodontal bone defects. For instance, Xiang et al86 designed a multifunctional nanohydroxyapatite assisted by tHA and modified the surface with BFP-1 and vascular endothelial QK via a single step of catechol chemistry, which was confirmed to regulate periodontal ligament stem cell activity. Moreover, Gao et al87 applied a similar strategy to prepare tHA/PCL with the purpose of cytocompatibility and osteogenesis enhancement, and their results indicated that the degradation rate and pore size would dominate the clinical application of the materials. Generally speaking, from 2D, 3D to 4D structures, mussel-based biomimetic materials present the advantages of toughness, resilience, and superior biocompatibility, suggesting a superior therapeutic effect on bone defects.
Osteosarcoma Treatment
Osteosarcoma (OS), frequently resulting in tumor-induced bone loss, is a prevailing disease that engenders extreme pain to patients.88,89 Currently, surgical resection, radiotherapy, and chemotherapy, as well as specific combinations of these treatments, are widely employed to eradicate osteosarcoma.89,90 However, healthy bone tissues are usually removed during the operation, which may lead to nonunion and fracture of the bone after surgery. Therefore, it is necessary to develop a bioactive implant to fill the defect, replace the removed bone tissue, and improve bone function recovery.91 To address these issues, Ma et al92 produced a 3D-printed bioceramic scaffold with a uniform self-assembled Ca-P/PDA nanolayer, which effectively induced tumor cell death and inhibited tumor growth. Notably, in previous works,93–95 a series of 3D scaffolds with bone regeneration and tumor inhibition capability was achieved by using photothermal agents, such as magnesium powder, Cu-TCPP nanosheets, or MoS2 nanosheets. In striking contrast, Jiang et al96 fabricated a mussel-inspired 3D-printed implant that released anticancer drugs and growth factors for enhanced anticancer treatment and osteogenesis over others through a PDA-assisted layer-by-layer assembly strategy. pZIF-8 (a nanoMOF), induced by mussels and pHA nanoparticles, was alternately assembled on the surface of 3D-printed gelatin scaffolds, manifesting that the mussel-biomimetic nanoMOF acted as a valid drug carrier and nano-building block in surface modification. Therefore, mussel nanostructures via 3D printing display promising potential in tumor therapy and bone regeneration, especially for tumor-induced bone tissue defects.
Osteoarthritis Treatment
Osteoarthritis (OA) is a common joint disease accompanied by pain and disability that arouses tremendous psychological and physiological stress in patients while posing a great burden on socioeconomic costs.97 Currently, tissue engineering, with the potential to overcome the defects of existing clinical treatment methods (disintegration of cartilage), has been well studied with great clinical potential.98 Moreover, mussel-based biomimetic materials are tailored to provide instructive cues for OA, which is an indispensable in tissue engineering.99 Mussels, containing a large amount of 12% glycosaminoglycans, are a high source of a rare, potent form of omega-3 fatty acids (called eicosatetraenoic acid (ETA)), indicating the intense anti-inflammatory properties through inhibiting the enzyme-related pathways (cyclooxygenase and lipoxygenase-based paths) from producing inflammatory factors.100 Interestingly, antioxidants in green-lipped mussels were proven to mediate the free radicals, which were the typical indicators in inflamed joints.101,102 Therefore, a variety of injectable materials have been developed to fill the joint cavity, capable of inflammatory factor elimination especially in the liquid form. For instance, Chen’s103 study demonstrated that GelMA hydrogel implantation combined with CM-SIN injection was a promising strategy for inducing autophagy and ameliorating osteoarthritis cartilage degradation. Furthermore, inspired by catecholamines, Han et al104 developed an injectable hydrogel microsphere for OA treatment by utilizing the lubrication characteristics of mussel cartilage through hydration lubrication of zwitterionic phosphobile base groups in the copolymer to synergistically reduce the coefficient of friction (Figure 5), which could also improve drug (diclofenac sodium) unloading via adjusting the proportion of dense coating on the surface of the microspheres.
![]() |
Figure 5 Mussel biomimetic material applied in osteoarthritis. (A) Schematic illustration of preparation process and application of [email protected] (B) In vitro cell viability and cell cytotoxicity of three different GelMA microspheres. (C) The results from H&E staining and Safranin O-fast green staining verified that functionalized GelMA microspheres can delay the progression of osteoarthritis in vivo. Reproduced with permission from: Han, Y., et al. Biomimetic injectable hydrogel microspheres with enhanced lubrication and controllable drug release for the treatment of osteoarthritis. Bioact Mater. 2021;6(10):3596–3607. doi: 10.1016/j.bioactmat.2021.03.022.104 Copyright 2021, Elsevier. https://creativecommons.org/licenses/by-nc-nd/4.0/. #Represent P < 0.05 by comparing with the control and blank groups, respectively. Abbreviation: NS, no significance. |
In addition, Bai et al105 designed a mussel-inspired peptide bound to the Ti-based implant, remarkably increasing the bone-implant contact area and improving osteogenesis. Besides, Xiao et al106 presented a surface engineering strategy to create functional surfaces by introducing dibenzylcyclooctyne (DBCO)-modified bioactive molecules on diverse surfaces. Similarly, Mou et al107 applied an analogous strategy to build a surface with DBCO-modified antimicrobial peptide (DBCO-AMP), indicating great potential for thrombosis and infection treatments. Collectively, no matter it is an injection system, an implant, or a surface coating, mussel-based materials exhibited promising potential osteoarthritis treatment by offering omega-3, unsaturated fatty acids and other anti-inflammatory substances.
Ligament Rupture Treatment
Ligament rupture mainly occurs in sports or an accident, requiring reconstruction of the injured ligament.108–110 To avoid complications resulting from autologous or allogeneic tendon transplantation, artificial ligament transplantation is an appropriate selection for human ligament reconstruction,111–113 which allows the patient to quickly recover and restore to normal moving status due to the excellent strength and toughness of artificial ligaments.114 Compared to natural ligaments, the bionic structure was challenged to induce the anterior cruciate ligament on the original interface.115,116 Inspired by mussels, Li et al117 successfully prepared biomimetic calcium phosphate APA/PDA-PET grafts with an active interface (Figure 6). Based on the synergistic effect of polyamines and apatite, the in vivo osseointegration during ligament reconstruction would be significantly improved, upon osteogenesis stimulation. However, direct graft-bone contact was not effective in reducing stress concentration at the interface compared to natural tendon-bone implantation. Fortunately, Yuan et al118 developed the CMWAs to enable the osteotendon interface reconstruction in the anterior cruciate ligament (ACL). In summary, mussel-based biomaterials could be well adjusted to fit the bone and joint interface by providing a superior adhesion to achieve joint ligament reconstruction.
![]() |
Figure 6 Mussel-based biomimetic material applied in ligament rupture. (A) Schematic illustration for mussel-inspired APA/PDA−PET grafts in vitro and in vivo experimental results. (B) The micro-CT images of the bone tunnels and the quantitative analysis in vivo promote the osseointegration of APA/PDA−PET Grafts. (C) The histological results of HE staining and Masson trichrome staining with quantitative analysis verified the results. Reprinted with permission from: Li H, Chen S, Chen J, et al. Mussel-inspired artificial grafts for functional ligament reconstruction. ACS Appl Mater Interfaces. 2015;7(27):14708–14719. doi:10.1021/acsami.5b05109.117 Copyright 2015, American Chemical Society. < 0.01), #p < 0.05. |
Osteoporosis Treatment
Osteoporosis is a global bone tissue disease that poses a heavy financial burden on the world’s health system, and the number of affected patients is expected to double in the next 20 years.119–121 Especially in older women, the risk and prevalence of the disease are significantly higher due to quicker bone tissue degradation, which may increase bone fragility and fracture.120 Facing this problem, PME-1 extracted from mussel byssus protein has been applied to promote the proliferation and differentiation of osteoblasts, indicating preventing effect against osteoporosis.122 In the future, unraveling the structure and function of this protein may help doctors and scientists become more potential candidates.
Conclusions and Outlook
Within the framework of this paper, we discussed the classification and adhesion mechanism of Mfp. To deepen the understanding of mussel-based biomimetic strategies in MSDs, we first introduced the different molecular structures related to mussel components, such as polysaccharides and peptides, and then discussed the existing synthesis methods. Finally, the specific applications, and the advantages and disadvantages of mussel biomimetic strategies in different diseases were included. It is believed that biomimetic adhesion materials have made outstanding achievements in scientific research and clinical applications, especially in the field of MSD treatment.123–125 Despite remarkable success in this area, there are still several challenges that need to be addressed. First, compared with natural methods, biomimetic approaches are not able to effectively regulate the oxidation reaction of DOPA or catechol in an alkaline environment. Second, considering the Mfp and other excellent adhesion properties of the material for multivariate equipping, the modification of free groups on the membrane surface can make biomaterials acquire new functions to make up for the deficiency of existing materials, research and development of the new type of high adhesion polymers is a future development trend of the bionic adhesion material. In this emerging area, it is obvious that geometric properties of mussel-based materials play a significant role in regulating their properties. Powered by the advantages of hierarchical architectures, advanced processing techniques such as 3D/4D printing offer great opportunities to organize mussel-based biomimetic materials to multiscale hierarchical structures, possibly extending their biomedical applications.
Acknowledgments
This study was supported by grants from the National Natural Science Foundation of China (81974348, 81772391) and Natural Science Foundation of Hubei Province (2022CFA063).
Disclosure
The authors declare no conflicts of interest in this work.
References
1. Zhang C, Wu B, Zhou Y, et al. Mussel-inspired hydrogels: from design principles to promising applications. Chem Soc Rev. 2020;49(11):3605–3637. doi:10.1039/c9cs00849g
2. Li J, Mooney DJ. Designing hydrogels for controlled drug delivery. Nat Rev Mater. 2016;1(12). doi:10.1038/natrevmats.2016.71
3. Chaudhuri O, Gu L, Klumpers D, et al. Hydrogels with tunable stress relaxation regulate stem cell fate and activity. Nat Mater. 2016;15(3):326–334. doi:10.1038/nmat4489
4. Ghobril C, Grinstaff MW. The chemistry and engineering of polymeric hydrogel adhesives for wound closure: a tutorial. Chem Soc Rev. 2015;44(7):1820–1835. doi:10.1039/c4cs00332b
5. Rich SI, Wood RJ, Majidi C. Untethered soft robotics. Nature Electr. 2018;1(2):102–112. doi:10.1038/s41928-018-0024-1
6. Yang C, Suo Z. Hydrogel ionotronics. Nature Rev Mater. 2018;3(6):125–142. doi:10.1038/s41578-018-0018-7
7. Yuk H, Lu B, Zhao X. Hydrogel bioelectronics. Chem Soc Rev. 2019;48(6):1642–1667. doi:10.1039/C8CS00595H
8. Wang Y, Zhu Y, Hu Y, et al. How to construct DNA hydrogels for environmental applications: advanced water treatment and environmental analysis. Small. 2018;14(17):e1703305. doi:10.1002/smll.201703305
9. Du Y, Zhang C, Zhong Q-Z, et al. Ultrathin alginate coatings as selective layers for nanofiltration membranes with high performance. ChemSusChem. 2017;10(13):2788–2795. doi:10.1002/cssc.201700519
10. Gao S, Sun J, Liu P, et al. A robust polyionized hydrogel with an unprecedented underwater anti-crude-oil-adhesion property. Adv Mater. 2016;28(26):5307–5314. doi:10.1002/adma.201600417
11. Zhao F, Bae J, Zhou X, Guo Y, Yu G. Nanostructured functional hydrogels as an emerging platform for advanced energy technologies. Adv Mater. 2018;30(48):e1801796. doi:10.1002/adma.201801796
12. Bae J, Li Y, Zhang J, et al. A 3D nanostructured hydrogel-framework-derived high-performance composite polymer lithium-ion electrolyte. Angew Chem Int Ed Engl. 2018;57(8):2096–2100. doi:10.1002/anie.201710841
13. Han L, Lu X, Liu K, et al. Mussel-inspired adhesive and tough hydrogel based on nanoclay confined dopamine polymerization. ACS Nano. 2017;11(3):2561–2574. doi:10.1021/acsnano.6b05318
14. Shao C, Wang M, Meng L, et al. Mussel-inspired cellulose nanocomposite tough hydrogels with synergistic self-healing, adhesive, and strain-sensitive properties. Chem Mater. 2018;30(9):3110–3121. doi:10.1021/acs.chemmater.8b01172
15. Li L, Yan B, Yang J, et al. Novel mussel-inspired injectable self-healing hydrogel with anti-biofouling property. Adv Mater. 2015;27(7):1294–1299. doi:10.1002/adma.201405166
16. He X, Liu L, Han H, et al. Bioinspired and microgel-tackified adhesive hydrogel with rapid self-healing and high stretchability. Macromolecules. 2019;52(1):72–80. doi:10.1021/acs.macromol.8b01678
17. Balestri W, Morris RH, Hunt JA, et al. Current advances on the regeneration of musculoskeletal interfaces. Tissue Eng Part B Rev. 2021;27(6):548–571. doi:10.1089/ten.TEB.2020.0112
18. Storheim K, Zwart JA. Musculoskeletal disorders and the Global Burden Of Disease study. Ann Rheum Dis. 2014;73(6):949–950. doi:10.1136/annrheumdis-2014-205327
19. Vos T, Flaxman AD, Naghavi M, et al. Years lived with disability (YLDs) for 1160 sequelae of 289 diseases and injuries 1990–2010: a systematic analysis for the Global Burden of Disease Study 2010. Lancet. 2012;380(9859):2163–2196. doi:10.1016/s0140-6736(12)61729-2
20. Babatunde OO, Jordan JL, Van der Windt DA, et al. Effective treatment options for musculoskeletal pain in primary care: a systematic overview of current evidence. PLoS One. 2017;12(6):e0178621. doi:10.1371/journal.pone.0178621
21. Lu HH, Subramony SD, Boushell MK, et al. Tissue engineering strategies for the regeneration of orthopedic interfaces. Ann Biomed Eng. 2010;38(6):2142–2154. doi:10.1007/s10439-010-0046-y
22. Liu W, Lipner J, Xie J, et al. Nanofiber scaffolds with gradients in mineral content for spatial control of osteogenesis. ACS Appl Mater Interfaces. 2014;6(4):2842–2849. doi:10.1021/am405418g
23. Smith BD, Grande DA. The current state of scaffolds for musculoskeletal regenerative applications. Nat Rev Rheumatol. 2015;11(4):213–222. doi:10.1038/nrrheum.2015.27
24. Shokri M, Dalili F, Kharaziha M, et al. Strong and bioactive bioinspired biomaterials, next generation of bone adhesives. Adv Colloid Interface Sci. 2022;305:102706. doi:10.1016/j.cis.2022.102706
25. Wu H, Zhao C, Lin K, et al. Mussel-inspired polydopamine-based multilayered coatings for enhanced bone formation. Front Bioeng Biotechnol. 2022;10:952500. doi:10.3389/fbioe.2022.952500
26. Harrington MJ, Jehle F, Priemel T. Mussel byssus structure-function and fabrication as inspiration for biotechnological production of advanced materials. Biotechnol J. 2018;13(12):e1800133. doi:10.1002/biot.201800133
27. Waite JH. The formation of mussel byssus: anatomy of a natural manufacturing process. Results Probl Cell Differ. 1992;19:27–54. doi:10.1007/978-3-540-47207-0_2
28. Clancy SK, Sodano A, Cunningham DJ, et al. Marine bioinspired underwater contact adhesion. Biomacromolecules. 2016;17(5):1869–1874. doi:10.1021/acs.biomac.6b00300
29. Tamarin A, Keller PJ. An ultrastructural study of the byssal thread forming system in Mytilus. J Ultrastruct Res. 1972;40(3):401–416. doi:10.1016/s0022-5320(72)90110-4
30. Zuccarello LV. The collagen gland of Mytilus galloprovincialis: an ultrastructural and cytochemical study on secretory granules. J Ultrastruct Res. 1980;73(2):135–147. doi:10.1016/s0022-5320(80)90119-7
31. Gim Y, Hwang DS, Lim S, et al. Production of fusion mussel adhesive fp-353 in Escherichia coli. Biotechnol Prog. 2008;24(6):1272–1277. doi:10.1002/btpr.65
32. Waite JH. Mussel adhesion – essential footwork. J Exp Biol. 2017;220(Pt 4):517–530. doi:10.1242/jeb.134056
33. Hwang DS, Gim Y, Cha HJ. Expression of functional recombinant mussel adhesive protein type 3A in Escherichia coli. Biotechnol Prog. 2005;21(3):965–970. doi:10.1021/bp050014e
34. Zhang W, Yang H, Liu F, et al. Molecular interactions between DOPA and surfaces with different functional groups: a chemical force microscopy study. RSC Adv. 2017;7(52):32518–32527. doi:10.1039/C7RA04228K
35. Aich P, An J, Yang B, et al. Self-assembled adhesive biomaterials formed by a genetically designed fusion protein. Chem Commun. 2018;54(89):12642–12645. doi:10.1039/c8cc07475e
36. Guo Q, Chen J, Wang J, et al. Recent progress in synthesis and application of mussel-inspired adhesives. Nanoscale. 2020;12(3):1307–1324. doi:10.1039/c9nr09780e
37. Kim HJ, Yang B, Park TY, et al. Complex coacervates based on recombinant mussel adhesive proteins: their characterization and applications. Soft Matter. 2017;13(42):7704–7716. doi:10.1039/c7sm01735a
38. Li Y, Cao Y. The molecular mechanisms underlying mussel adhesion. Nanoscale Adv. 2019;1(11):4246–4257. doi:10.1039/C9NA00582J
39. Silverman HG, Roberto FF. Understanding marine mussel adhesion. Mar Biotechnol. 2007;9(6):661–681. doi:10.1007/s10126-007-9053-x
40. Yang J, Cohen Stuart MA, Kamperman M. Jack of all trades: versatile catechol crosslinking mechanisms. Chem Soc Rev. 2014;43(24):8271–8298. doi:10.1039/c4cs00185k
41. Shin M, Shin JY, Kim K, et al. The position of lysine controls the catechol-mediated surface adhesion and cohesion in underwater mussel adhesion. J Colloid Interface Sci. 2020;563:168–176. doi:10.1016/j.jcis.2019.12.082
42. Lee H, Dellatore SM, Miller WM, et al. Mussel-inspired surface chemistry for multifunctional coatings. Science. 2007;318(5849):426–430. doi:10.1126/science.1147241
43. Yu J, Wei W, Menyo MS, et al. Adhesion of mussel foot Protein-3 to TiO 2 surfaces: the effect of pH. Biomacromolecules. 2013;14(4):1072–1077. doi:10.1021/bm301908y
44. Hansen DC, Zimlich KR, Bennett BN. Inhibition of flash rusting of HY80 by a mussel adhesive protein: characterizing the interaction of MeFP-5 with a high strength low alloy steel. Electrochim Acta. 2019;301:411–420. doi:10.1016/j.electacta.2019.01.145
45. Hong JM, Kim BJ, Shim J-H, et al. Enhancement of bone regeneration through facile surface functionalization of solid freeform fabrication-based three-dimensional scaffolds using mussel adhesive proteins. Acta Biomater. 2012;8(7):2578–2586. doi:10.1016/j.actbio.2012.03.041
46. Choi YS, Kang DG, Lim S, et al. Recombinant mussel adhesive protein fp-5 (MAP fp-5) as a bulk bioadhesive and surface coating material. Biofouling. 2011;27(7):729–737. doi:10.1080/08927014.2011.600830
47. Waite JH. Reverse engineering of bioadhesion in marine mussels. Ann N Y Acad Sci. 1999;875:301–309. doi:10.1111/j.1749-6632.1999.tb08513.x
48. Lee H, Lee KD, Pyo KB, et al. Catechol-grafted poly(ethylene glycol) for PEGylation on versatile substrates. Langmuir. 2010;26(6):3790–3793. doi:10.1021/la904909h
49. Qu J, Zhao X, Liang Y, et al. Antibacterial adhesive injectable hydrogels with rapid self-healing, extensibility and compressibility as wound dressing for joints skin wound healing. Biomaterials. 2018;183:185–199. doi:10.1016/j.biomaterials.2018.08.044
50. Kim E, Dai B, Qiao JB, et al. Microbially synthesized repeats of mussel foot protein display enhanced underwater adhesion. ACS Appl Mater Interfaces. 2018;10(49):43003–43012. doi:10.1021/acsami.8b14890
51. Wei Q, Achazi K, Liebe H, et al. Mussel-inspired dendritic polymers as universal multifunctional coatings. Angew Chem Int Ed Engl. 2014;53(43):11650–11655. doi:10.1002/anie.201407113
52. Numata K, Baker PJ. Synthesis of adhesive peptides similar to those found in blue mussel (Mytilus edulis) using papain and tyrosinase. Biomacromolecules. 2014;15(8):3206–3212. doi:10.1021/bm5009052
53. Suci PA, Geesey GG. Influence of sodium periodate and tyrosinase on binding of alginate to adlayers of Mytilus edulis foot protein 1. J Colloid Interface Sci. 2000;230(2):340–348. doi:10.1006/jcis.2000.7120
54. Huang Q, Zhao J, Liu M, et al. Preparation of polyethylene [email protected] acid encapsulated MgAl-layered double hydroxide for the efficient removal of copper (II) ions from aqueous solution. J Taiwan Inst Chem Eng. 2018;82:92–101. doi:10.1016/j.jtice.2017.10.019
55. Yamamoto H, Kuno S, Nagai A, et al. Insolubilizing and adhesive studies of water-soluble synthetic model proteins. Int J Biol Macromol. 1990;12(5):305–310. doi:10.1016/0141-8130(90)90019-7
56. Yamamoto H, Ito K, Ishiguro S-I, et al. Gene controlling a differentiation step in the quail melanocyte. Dev Genet. 1987;8(3):179–185. doi:10.1002/dvg.1020080306
57. Wang J, Liu C, Lu X, et al. Co-polypeptides of 3,4-dihydroxyphenylalanine and L-lysine to mimic marine adhesive protein. Biomaterials. 2007;28(23):3456–3468. doi:10.1016/j.biomaterials.2007.04.009
58. Yi Y, Xu W, Wang H-X, et al. Natural polysaccharides experience physiochemical and functional changes during preparation: a review. Carbohydr Polym. 2020;234:115896. doi:10.1016/j.carbpol.2020.115896
59. Samyn P. A platform for functionalization of cellulose, chitin/ chitosan, alginate with polydopamine: a review on fundamentals and technical applications. Int J Biol Macromol. 2021;178:71–93. doi:10.1016/j.ijbiomac.2021.02.091
60. Fu Y, Yang L, Zhang J, et al. Polydopamine antibacterial materials. Mater Horiz. 2021;8(6):1618–1633. doi:10.1039/d0mh01985b
61. Lee HA, Park E, Lee H. Polydopamine and its derivative surface chemistry in material science: a focused review for studies at KAIST. Adv Mater. 2020;32(35):e1907505. doi:10.1002/adma.201907505
62. Fangfei Liu XL, Chen F, Fu Q. Mussel-inspired chemistry: a promising strategy for natural polysaccharides in biomedical applications. Prog Polym Sci. 2021;123:101472.
63. Ryu JH, Hong S, Lee H. Bio-inspired adhesive catechol-conjugated chitosan for biomedical applications: a mini review. Acta Biomater. 2015;27:101–115. doi:10.1016/j.actbio.2015.08.043
64. Hu Y, Zhan C, Zhou A, Zhang S, Chen J, Huang X. Synthesis and characterization of L-tyrosine-conjugated quaternary ammonium salt chitosan and their cytocompatibility as a potential tissue engineering scaffold. J Biomater Sci Polym Ed. 2020;31(7):833–848. doi:10.1080/09205063.2020.1712174
65. Zhang Y, Thomas Y, Kim E, et al. pH- and voltage-responsive chitosan hydrogel through covalent cross-linking with catechol. J Phys Chem B. 2012;116(5):1579–1585. doi:10.1021/jp210043w
66. Hong S, Lee H, Lee H. Controlling mechanical properties of bio-inspired hydrogels by modulating nano-scale, inter-polymeric junctions. Beilstein J Nanotechnol. 2014;5:887–894. doi:10.3762/bjnano.5.101
67. Berger O, Battistella C, Chen Y, et al. Mussel adhesive-inspired proteomimetic polymer. J Am Chem Soc. 2022;144(10):4383–4392. doi:10.1021/jacs.1c10936
68. Han L, Liu K, Wang M, et al. Mussel-inspired adhesive and conductive hydrogel with long-lasting moisture and extreme temperature tolerance. Adv Funct Mater. 2018;28(3):1704195. doi:10.1002/adfm.201704195
69. Zheng K, Gu Q, Zhou D, Zhou M, Zhang L. Recent progress in surgical adhesives for biomedical applications. Smart Mater Med. 2022;3:41–65. doi:10.1016/j.smaim.2021.11.004
70. Bentley G, Bhamra JS, Gikas PD, et al. Repair of osteochondral defects in joints–how to achieve success. Injury. 2013;44(Suppl 1):S3–S10. doi:10.1016/s0020-1383(13)70003-2
71. Xu X, Chen X, Li J. Natural protein bioinspired materials for regeneration of hard tissues. J Mater Chem B. 2020;8(11):2199–2215. doi:10.1039/d0tb00139b
72. Liu Y, Ai K, Lu L. Polydopamine and its derivative materials: synthesis and promising applications in energy, environmental, and biomedical fields. Chem Rev. 2014;114(9):5057–5115. doi:10.1021/cr400407a
73. Park JP, Song IT, Lee J, et al. Vanadyl–catecholamine hydrogels inspired by ascidians and mussels. Chem Mater. 2015;27(1):105–111. doi:10.1021/cm503425d
74. Gan D, Xu T, Xing W, et al. Mussel-inspired dopamine oligomer intercalated tough and resilient gelatin methacryloyl (GelMA) hydrogels for cartilage regeneration. J Mater Chem B. 2019;7(10):1716–1725. doi:10.1039/c8tb01664j
75. Han L, Wang M, Li P, et al. Mussel-inspired tissue-adhesive hydrogel based on the polydopamine-chondroitin sulfate complex for growth-factor-free cartilage regeneration. ACS Appl Mater Interfaces. 2018;10(33):28015–28026. doi:10.1021/acsami.8b05314
76. Ju X, Liu X, Zhang Y, et al. A photo-crosslinked proteinogenic hydrogel enabling self-recruitment of endogenous TGF-β1 for cartilage regeneration. Smart Mater Med. 2022;3:85–93. doi:10.1016/j.smaim.2021.12.002
77. Gan D, Wang Z, Xie C, et al. Mussel-inspired tough hydrogel with in situ nanohydroxyapatite mineralization for osteochondral defect repair. Adv Healthc Mater. 2019;8(22):e1901103. doi:10.1002/adhm.201901103
78. Giannoni P, Lazzarini E, Ceseracciu L, et al. Design and characterization of a tissue-engineered bilayer scaffold for osteochondral tissue repair. J Tissue Eng Regen Med. 2015;9(10):1182–1192. doi:10.1002/term.1651
79. Liu X, Chen W, Shao B, et al. Mussel patterned with 4D biodegrading elastomer durably recruits regenerative macrophages to promote regeneration of craniofacial bone. Biomaterials. 2021;276:120998. doi:10.1016/j.biomaterials.2021.120998
80. Chen FM, Jin Y. Periodontal tissue engineering and regeneration: current approaches and expanding opportunities. Tissue Eng Part B Rev. 2010;16(2):219–255. doi:10.1089/ten.TEB.2009.0562
81. Izumi Y, Aoki A, Yamada Y, et al. Current and future periodontal tissue engineering. Periodontol. 2011;56(1):166–187. doi:10.1111/j.1600-0757.2010.00366.x
82. Li G, Zhou T, Lin S, et al. Nanomaterials for craniofacial and dental tissue engineering. J Dent Res. 2017;96(7):725–732. doi:10.1177/0022034517706678
83. Valerio MS, Alexis F, Kirkwood KL. Functionalized nanoparticles containing MKP-1 agonists reduce periodontal bone loss. J Periodontol. 2019;90(8):894–902. doi:10.1002/jper.18-0572
84. Ni C, Zhou J, Kong N, et al. Gold nanoparticles modulate the crosstalk between macrophages and periodontal ligament cells for periodontitis treatment. Biomaterials. 2019;206:115–132. doi:10.1016/j.biomaterials.2019.03.039
85. Aminu N, Chan S-Y, Yam M-F, et al. A dual-action chitosan-based nanogel system of triclosan and flurbiprofen for localised treatment of periodontitis. Int J Pharm. 2019;570:118659. doi:10.1016/j.ijpharm.2019.118659
86. Xiang M, Zhu M, Yang Z, et al. Dual-functionalized apatite nanocomposites with enhanced cytocompatibility and osteogenesis for periodontal bone regeneration. ACS Biomater Sci Eng. 2020;6(3):1704–1714. doi:10.1021/acsbiomaterials.9b01893
87. Gao X, Song J, Ji P, et al. Polydopamine-templated hydroxyapatite reinforced polycaprolactone composite nanofibers with enhanced cytocompatibility and osteogenesis for bone tissue engineering. ACS Appl Mater Interfaces. 2016;8(5):3499–3515. doi:10.1021/acsami.5b12413
88. Randall RL. A promise to our patients with metastatic bone disease. Ann Surg Oncol. 2014;21(13):4049–4050. doi:10.1245/s10434-014-4010-1
89. Zhang K, Zhou Y, Xiao C, et al. Application of hydroxyapatite nanoparticles in tumor-associated bone segmental defect. Sci Adv. 2019;5(8):eaax6946. doi:10.1126/sciadv.aax6946
90. Ferguson JL, Turner SP. Bone cancer: diagnosis and treatment principles. Am Fam Physician. 2018;98(4):205–213.
91. Ogose A, Hotta T, Kawashima H, et al. Comparison of hydroxyapatite and beta tricalcium phosphate as bone substitutes after excision of bone tumors. J Biomed Mater Res B Appl Biomater. 2005;72(1):94–101. doi:10.1002/jbm.b.30136
92. Ma H, Luo J, Sun Z, et al. 3D printing of biomaterials with mussel-inspired nanostructures for tumor therapy and tissue regeneration. Biomaterials. 2016;111:138–148. doi:10.1016/j.biomaterials.2016.10.005
93. Harrington MJ, Masic A, Holten-Andersen N, et al. Iron-clad fibers: a metal-based biological strategy for hard flexible coatings. Science. 2010;328(5975):216–220. doi:10.1126/science.1181044
94. Wang X, Xue J, Ma B, et al. Black bioceramics: combining regeneration with therapy. Adv Mater. 2020;32(48):e2005140. doi:10.1002/adma.202005140
95. Dang W, Ma B, Li B, et al. 3D printing of metal-organic framework nanosheets-structured scaffolds with tumor therapy and bone construction. Biofabrication. 2020;12(2):025005. doi:10.1088/1758-5090/ab5ae3
96. Jiang Y, Pan X, Yao M, et al. Bioinspired adhesive and tumor microenvironment responsive nanoMOFs assembled 3D-printed scaffold for anti-tumor therapy and bone regeneration. Nano Today. 2021;39:101182. doi:10.1016/j.nantod.2021.101182
97. Glyn-Jones S, Palmer AJR, Agricola R, et al. Osteoarthritis. Lancet. 2015;386(9991):376–387. doi:10.1016/s0140-6736(14)60802-3
98. Zhou Z, Cui J, Wu S, et al. Silk fibroin-based biomaterials for cartilage/osteochondral repair. Theranostics. 2022;12(11):5103–5124. doi:10.7150/thno.74548
99. Madhurakkat Perikamana SK, Lee J, Lee YB, et al. Materials from mussel-inspired chemistry for cell and tissue engineering applications. Biomacromolecules. 2015;16(9):2541–2555. doi:10.1021/acs.biomac.5b00852
100. Jhun J, Na HS, Cho K-H, et al. A green-lipped mussel reduces pain behavior and chondrocyte inflammation and attenuated experimental osteoarthritis progression. PLoS One. 2021;16(12):e0259130. doi:10.1371/journal.pone.0259130
101. Alghamdi AA, Al-Hazmi A, Almalki AA, et al. Antioxidant activity derived from marine green-lipped mussel perna canaliculus extracts in mice. Biomed Res Int. 2021;2021:1622270. doi:10.1155/2021/1622270
102. Calabrese G, Ardizzone A, Campolo M, et al. Beneficial effect of tempol, a membrane-permeable radical scavenger, on inflammation and osteoarthritis in in vitro models. Biomolecules. 2021;11(3):352. doi:10.3390/biom11030352
103. Chen P, Xia C, Mei S, et al. Intra-articular delivery of sinomenium encapsulated by chitosan microspheres and photo-crosslinked GelMA hydrogel ameliorates osteoarthritis by effectively regulating autophagy. Biomaterials. 2016;81:1–13. doi:10.1016/j.biomaterials.2015.12.006
104. Han Y, Yang J, Zhao W, et al. Biomimetic injectable hydrogel microspheres with enhanced lubrication and controllable drug release for the treatment of osteoarthritis. Bioact Mater. 2021;6(10):3596–3607. doi:10.1016/j.bioactmat.2021.03.022
105. Bai J, Wang H, Chen H, et al. Biomimetic osteogenic peptide with mussel adhesion and osteoimmunomodulatory functions to ameliorate interfacial osseointegration under chronic inflammation. Biomaterials. 2020;255:120197. doi:10.1016/j.biomaterials.2020.120197
106. Xiao Y, Wang W, Tian X, et al. A versatile surface bioengineering strategy based on mussel-inspired and bioclickable peptide mimic. Research. 2020;2020:7236946. doi:10.34133/2020/7236946
107. Mou X, Zhang H, Qiu H, et al. Mussel-inspired and bioclickable peptide engineered surface to combat thrombosis and infection. Research. 2022;2022:9780879. doi:10.34133/2022/9780879
108. Marrale J, Morrissey MC, Haddad FS. A literature review of autograft and allograft anterior cruciate ligament reconstruction. Knee Surg Sports Traumatol Arthrosc. 2007;15(6):690–704. doi:10.1007/s00167-006-0236-1
109. Musahl V, Karlsson J, Solomon CG. Anterior Cruciate Ligament Tear. N Engl J Med. 2019;380(24):2341–2348. doi:10.1056/NEJMcp1805931
110. Liu ZT, Zhang XL, Jiang Y, Zeng BF. Four-strand hamstring tendon autograft versus LARS artificial ligament for anterior cruciate ligament reconstruction. Int Orthop. 2010;34(1):45–49. doi:10.1007/s00264-009-0768-3
111. Parchi PD, Gianluca C, Dolfi L, et al. Anterior cruciate ligament reconstruction with LARS™ artificial ligament results at a mean follow-up of eight years. Int Orthop. 2013;37(8):1567–1574. doi:10.1007/s00264-013-1917-2
112. Shen G, Xu Y, Dong Q, Zhou H, Yu C. Arthroscopic posterior cruciate ligament reconstruction using LARS artificial ligament: a retrospective study. J Surg Res. 2012;173(1):75–82. doi:10.1016/j.jss.2010.08.015
113. Hamido F, Misfer AK, Al Harran H, et al. The use of the LARS artificial ligament to augment a short or undersized ACL hamstrings tendon graft. Knee. 2011;18(6):373–378. doi:10.1016/j.knee.2010.09.003
114. Ranger P, Renaud A, Phan P, et al. Evaluation of reconstructive surgery using artificial ligaments in 71 acute knee dislocations. Int Orthop. 2011;35(10):1477–1482. doi:10.1007/s00264-010-1154-x
115. Gao K, Chen S, Wang L, et al. Anterior cruciate ligament reconstruction with LARS artificial ligament: a multicenter study with 3- to 5-year follow-up. Arthroscopy. 2010;26(4):515–523. doi:10.1016/j.arthro.2010.02.001
116. Viateau V, Manassero M, Anagnostou F, et al. Biological and biomechanical evaluation of the ligament advanced reinforcement system (LARS AC) in a sheep model of anterior cruciate ligament replacement: a 3-month and 12-month study. Arthroscopy. 2013;29(6):1079–1088. doi:10.1016/j.arthro.2013.02.025
117. Li H, Chen S, Chen J, et al. Mussel-inspired artificial grafts for functional ligament reconstruction. ACS Appl Mater Interfaces. 2015;7(27):14708–14719. doi:10.1021/acsami.5b05109
118. Yuan X, Zhao Y, Li J, et al. Citrate-based mussel-inspired magnesium whitlockite composite adhesives augmented bone-to-tendon healing. J Mater Chem B. 2021;9(39):8202–8210. doi:10.1039/d1tb01710a
119. Harvey N, Dennison E, Cooper C. Osteoporosis: impact on health and economics. Nat Rev Rheumatol. 2010;6(2):99–105. doi:10.1038/nrrheum.2009.260
120. Eastell R, Szulc P. Use of bone turnover markers in postmenopausal osteoporosis. Lancet Diabetes Endocrinol. 2017;5(11):908–923. doi:10.1016/s2213-8587(17)30184-5
121. Coughlan T, Dockery F. Osteoporosis and fracture risk in older people. Clin Med. 2014;14(2):187–191. doi:10.7861/clinmedicine.14-2-187
122. Xu Z, Fan F, Chen H, et al. Absorption and transport of a Mytilus edulis -derived peptide with the function of preventing osteoporosis. Food Funct. 2021;12(5):2102–2111. doi:10.1039/d0fo02353a
123. Barros NR, Chen Y, Hosseini V, et al. Recent developments in mussel-inspired materials for biomedical applications. Biomater Sci. 2021;9(20):6653–6672. doi:10.1039/d1bm01126j
124. Lu M, Yu J. Mussel-inspired biomaterials for cell and tissue engineering. Adv Exp Med Biol. 2018;1077:451–474. doi:10.1007/978-981-13-0947-2_24
125. Yu L, Wei M. Biomineralization of collagen-based materials for hard tissue repair. Int J Mol Sci. 2021;22(2). doi:10.3390/ijms22020944
126. Casanellas I, García-Lizarribar A, Lagunas A, et al. Producing 3D biomimetic nanomaterials for musculoskeletal system regeneration. Front Bioeng Biotechnol. 2018;6:128. doi:10.3389/fbioe.2018.00128
127. Kim HJ, Hwang BH, Lim S, et al. Mussel adhesion-employed water-immiscible fluid bioadhesive for urinary fistula sealing. Biomaterials. 2015;72:104–111. doi:10.1016/j.biomaterials.2015.08.055
128. Hwang DS, Zeng H, Srivastava A, et al. Viscosity and interfacial properties in a mussel-inspired adhesive coacervate. Soft Matter. 2010;6(14):3232–3236. doi:10.1039/C002632H
129. Lim S, Choi YS, Kang DG, et al. The adhesive properties of coacervated recombinant hybrid mussel adhesive proteins. Biomaterials. 2010;31(13):3715–3722. doi:10.1016/j.biomaterials.2010.01.063
130. Balkenende DWR, Winkler SM, Messersmith PB. Marine-inspired polymers in medical adhesion. Eur Polym J. 2019;116:134–143. doi:10.1016/j.eurpolymj.2019.03.059
131. Hofman AH, van Hees IA, Yang J, et al. Bioinspired underwater adhesives by using the supramolecular toolbox. Adv Mater. 2018;30(19):e1704640. doi:10.1002/adma.201704640
132. Bhagat V, Becker ML. Degradable adhesives for surgery and tissue engineering. Biomacromolecules. 2017;18(10):3009–3039. doi:10.1021/acs.biomac.7b00969
133. Li L, Zeng H. Marine mussel adhesion and bio-inspired wet adhesives. Biotribology. 2016;5:44–51. doi:10.1016/j.biotri.2015.09.004
134. Li L, Smitthipong W, Zeng H. Mussel-inspired hydrogels for biomedical and environmental applications. Polym Chem. 2015;6(3):353–358. doi:10.1039/C4PY01415D
135. Carrington E, Waite JH, Sarà G, et al. Mussels as a model system for integrative ecomechanics. Ann Rev Mar Sci. 2015;7(1):443–469. doi:10.1146/annurev-marine-010213-135049
136. Kim S, Huang J, Lee Y, et al. Complexation and coacervation of like-charged polyelectrolytes inspired by mussels. Proc Natl Acad Sci U S A. 2016;113(7):E847–E853. doi:10.1073/pnas.1521521113
137. Miller DR, Das S, Huang K-Y, et al. Mussel coating protein-derived complex coacervates mitigate frictional surface damage. ACS Biomater Sci Eng. 2015;1(11):1121–1128. doi:10.1021/acsbiomaterials.5b00252
138. Brubaker CE, Messersmith PB. Enzymatically degradable mussel-inspired adhesive hydrogel. Biomacromolecules. 2011;12(12):4326–4334. doi:10.1021/bm201261d
139. Chen T, Chen Y, Rehman HU, et al. Ultratough, self-healing, and tissue-adhesive hydrogel for wound dressing. ACS Appl Mater Interfaces. 2018;10(39):33523–33531. doi:10.1021/acsami.8b10064
140. Krogsgaard M, Behrens MA, Pedersen JS, Birkedal H. Self-healing mussel-inspired multi-pH-responsive hydrogels. Biomacromolecules. 2013;14(2):297–301. doi:10.1021/bm301844u
141. Wilke P, Helfricht N, Mark A, Papastavrou G, Faivre D, Borner HG. A direct biocombinatorial strategy toward next generation, mussel-glue inspired saltwater adhesives. J Am Chem Soc. 2014;136(36):12667–12674. doi:10.1021/ja505413e
142. Han L, Yan L, Wang M, et al. Transparent, adhesive, and conductive hydrogel for soft bioelectronics based on light-transmitting polydopamine-doped polypyrrole nanofibrils. Chem Mater. 2018;30(16):5561–5572. doi:10.1021/acs.chemmater.8b01446
143. Kim M, Chung H. Photo-responsive bio-inspired adhesives: facile control of adhesion strength via a photocleavable crosslinker. Polym Chem. 2017;8(40):6300–6308. doi:10.1039/C7PY01535F
144. Yang B, Ayyadurai N, Yun H, et al. In vivo residue-specific dopa-incorporated engineered mussel bioglue with enhanced adhesion and water resistance. Angew Chem Int Ed Engl. 2014;53(49):13360–13364. doi:10.1002/anie.201406099
145. Zhan K, Kim C, Sung K, et al. Tunicate-inspired gallol polymers for underwater adhesive: a comparative study of catechol and gallol. Biomacromolecules. 2017;18(9):2959–2966. doi:10.1021/acs.biomac.7b00921
146. Wang W, Zhang Y, Liu W. Bioinspired fabrication of high strength hydrogels from non-covalent interactions. Prog Polym Sci. 2017;71:1–25. doi:10.1016/j.progpolymsci.2017.04.001
147. Anderson TH, Yu J, Estrada A, et al. The contribution of DOPA to substrate-peptide adhesion and internal cohesion of mussel-inspired synthetic peptide films. Adv Funct Mater. 2010;20(23):4196–4205. doi:10.1002/adfm.201000932
148. Utzig T, Stock P, Valtiner M. Resolving non-specific and specific adhesive interactions of catechols at solid/liquid interfaces at the molecular scale. Angew Chem Int Ed Engl. 2016;55(33):9524–9528. doi:10.1002/anie.201601881
149. Zhang C, Gong L, Xiang L, et al. Deposition and adhesion of polydopamine on the surfaces of varying wettability. ACS Appl Mater Interfaces. 2017;9(36):30943–30950. doi:10.1021/acsami.7b09774
150. Israelachvili J, Pashley R. The hydrophobic interaction is long range, decaying exponentially with distance. Nature. 1982;300(5890):341–342. doi:10.1038/300341a0
151. Ma JC, Dougherty DA. The cation minus sign pi interaction. Chem Rev. 1997;97(5):1303–1324. doi:10.1021/cr9603744
152. Chang G, Yang L, Yang J, et al. High-performance pH-switchable supramolecular thermosets via cation-π interactions. Adv Mater. 2018;30(7):1704234. doi:10.1002/adma.201704234
153. Lu Q, Oh DX, Lee Y, et al. Nanomechanics of cation-π interactions in aqueous solution. Angew Chem Int Ed Engl. 2013;52(14):3944–3948. doi:10.1002/anie.201210365
154. Maier GP, Rapp MV, Waite JH, et al. Biological Adhesives. Adaptive synergy between catechol and lysine promotes wet adhesion by surface salt displacement. Science. 2015;349(6248):628–632. doi:10.1126/science.aab0556
155. Rapp MV, Maier GP, Dobbs HA, et al. Defining the catechol-cation synergy for enhanced wet adhesion to mineral surfaces. J Am Chem Soc. 2016;138(29):9013–9016. doi:10.1021/jacs.6b03453
156. Sinnokrot MO, Sherrill CD. High-accuracy quantum mechanical studies of pi-pi interactions in benzene dimers. J Phys Chem A. 2006;110(37):10656–10668. doi:10.1021/jp0610416
157. Grimme S. Do special noncovalent pi-pi stacking interactions really exist? Angew Chem Int Ed Engl. 2008;47(18):3430–3434. doi:10.1002/anie.200705157
158. Kawase T, Kurata H. Ball-, bowl-, and belt-shaped conjugated systems and their complexing abilities: exploration of the concave-convex pi-pi interaction. Chem Rev. 2006;106(12):5250–5273. doi:10.1021/cr0509657
159. Li Y, Liao M, Zhou J. Catechol and its derivatives adhesion on graphene: insights from molecular dynamics simulations. J Phys Chem C. 2018;122(40):22965–22974. doi:10.1021/acs.jpcc.8b06392
160. Hwang DS, Zeng H, Masic A, et al. Protein- and metal-dependent interactions of a prominent protein in mussel adhesive plaques. J Biol Chem. 2010;285(33):25850–25858. doi:10.1074/jbc.M110.133157
161. Yan J, Springsteen G, Deeter S, et al. The relationship among pKa, pH, and binding constants in the interactions between boronic acids and diols—it is not as simple as it appears. Tetrahedron. 2004;60(49):11205–11209. doi:10.1016/j.tet.2004.08.051
162. Zhao X, Zhang M, Guo B, et al. Mussel-inspired injectable supramolecular and covalent bond crosslinked hydrogels with rapid self-healing and recovery properties via a facile approach under metal-free conditions. J Mater Chem B. 2016;4(41):6644–6651. doi:10.1039/c6tb01776b
163. Lee Y, Jun K, Lee K, et al. Phenol-derived carbon sealant inspired by a coalification process. Angew Chem Int Ed Engl. 2020;59(10):3864–3870. doi:10.1002/anie.201913181
164. Byun E, Ryu JH, Lee H. Catalyst-mediated yet catalyst-free hydrogels formed by interfacial chemical activation. Chem Commun. 2014;50(22):2869–2872. doi:10.1039/c3cc49043b
165. Zhang FX, Liu P, Ding W, et al. Injectable Mussel-Inspired highly adhesive hydrogel with exosomes for endogenous cell recruitment and cartilage defect regeneration. Biomaterials. 2021;278:121169. doi:10.1016/j.biomaterials.2021.121169