Nanotherapeutic and Stem Cell Therapeutic Strategies in Neurodegenerative Diseases: A Promising Therapeutic Approach
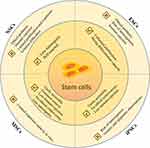
Introduction
Neurodegenerative diseases are characterized by the formation of disease-specific misfolded proteins, progressive neuronal degeneration and loss, and, ultimately, they lead to cognitive and/or sensorimotor dysfunction. They include Alzheimer’s disease (AD), Parkinson’s disease (PD), Huntington’s disease (HD), amyotrophic lateral sclerosis (ALS), and multiple system atrophy (MSA).1–3 In these diseases, some specific neuronal subsets, such as dopaminergic neurons, motor neurons, or cholinergic neurons, gradually lose their structure, function, or number, leading to specific types of nervous system dysfunctions.4,5 Therapeutic progress in neurodegenerative diseases remains slow due to insufficient understanding of disease pathogenesis and the difficulty of drugs crossing the blood–brain barrier (BBB).6–8 Currently available treatments for neurodegenerative diseases can only alleviate symptoms and are not sufficient to prevent neurodegenerative processes. Therefore, effective treatments are urgently needed.
Among various therapeutic approaches, stem cell therapy is considered as one of the most promising approaches for the treatment of neurodegenerative diseases.5,9,10 Stem cell-based therapeutic strategies mainly include directly replacing damaged cells in interested tissues by transplanting exogenous stem cells or indirectly promoting the renewal of damaged nerve tissues by activating endogenous neural progenitor cells, as well as autocrine/paracrine effects.11,12 However, stem cell therapy is hampered by critical issues such as poor differentiation and survival after cell transplantation, insufficient homing of stem cells to the injured site, and the inability to track and monitor the injected/transplanted stem cells in vivo.13–15
With the development of nanotechnology, nanomaterials hold great promise in the treatment of neurodegenerative diseases due to their unique properties, such as high surface area-to-volume ratios, tunable surface chemistry, enhanced electrical conductivity, high mechanical strength, unique fluorescence and superparamagnetic properties.16–18 These unique properties enable some nanomaterials to combat oxidative stress and scavenge abnormally aggregated proteins.19–21 Meanwhile, since nanomaterials can cross the BBB, they are ideal stem cell/drug/gene carriers to achieve targeted delivery to the brain and even allow noninvasive tracking by imaging techniques.6,22–24 Furthermore, engineered nanomaterial-based scaffolds can mimic the stem cell microenvironment to improve stem cell survival and promote their differentiation into neurons.13,25 Therefore, the cooperation of nanotherapeutic and stem cell therapeutic strategies can improve the therapeutic effect of neurodegenerative diseases and obtain better clinical benefits. Accordingly, this review focuses on recent advances and systematically summarizes the applications and challenges of nanotherapeutics combined with stem cell therapy for the treatment of neurodegenerative diseases.
Stem Cell Therapeutic Strategies
Stem cells are a class of cells that can proliferate, self-renew, and differentiate into various mature cell lines. Stem cell therapy has been used to treat neurodegenerative diseases for decades, especially for the treatment of PD, with considerable success in various cell transplantation studies.26–28 Stem cell therapeutic strategies for neurodegenerative diseases mainly include direct replacement of damaged or lost cells, secretion of neurotrophic and growth factors by autocrine/paracrine effects, or activation of endogenous neural precursor cells.11,29,30 Endogenous neural precursor cells are usually poorly differentiated; exogenous stem cells promote their proliferation and differentiation and restore cell survival microenvironments, enabling activation of endogenous neural precursor cells.29 The transplanted cells provide a variety of bioactive exosomes, neurotrophic factors, and cytokines that play important therapeutic roles for the injured nerve tissue by self-secretion as well as promoting the secretion of peripheral nerve cells, such as nerve growth factor (NGF), neurotrophin-3 (NT3), brain-derived neurotrophic factor (BDNF), glial-derived neurotrophic factor (GDNF), and insulin growth factor (IGF).31–33 These secreted bioactive products can promote endogenous neuronal growth, stimulate neurogenesis, decrease apoptosis, regulate inflammation, and encourage synaptic connection from damaged neurons.11,34 Furthermore, stem cells have a homing effect: they can recognize and migrate to the injury or lesion site and then generate new neural cells to repair the injury.35,36
At present, embryonic stem cells (ESCs), neural stem cells (NSCs), induced pluripotent stem cells (iPSCs), and mesenchymal stem cells (MSCs) are the main stem cell types used in the treatment of neurodegenerative diseases.11,29,37 As shown in Figure 1, the benefits and limitations of these stem cells in basic research and preclinical trials are summarized. Each type of stem cell has its particular advantages and disadvantages, which require understanding the characteristics of the various stem cell types and their rational design based on the desired application and outcome.5,38
![]() |
Figure 1 Advantages and limitations of various types of stem cells in the treatment of neurodegenerative diseases. |
Nanotherapeutic Strategies
Nanotherapeutic strategies increasingly play an important role in the diagnosis and treatment of neurodegenerative diseases in recent years.39,40 Various novel nanomaterials are fabricated for the treatment of neurodegenerative diseases (Figure 2). Drug treatment of neurodegenerative diseases often fails due to the poor permeability of compounds to the BBB, which functions as a defense system and prevents the penetration of most drugs, while nanomaterials can pass through.8,15 Therefore, nanomaterials are often fabricated as carriers for drugs/genes/cells. In order to obtain “intelligence” nano-drug delivery systems, functionalized targeting ligands (eg, RGD Peptides, transferrin, insulin) can confer targeting specificity to nanocarriers.41 In addition, the controlled release of loaded drugs can be achieved by self-degradation of the carrier, or by internal or external stimuli (eg, redox, pH, temperature, magnetic field, light, etc.), thereby minimizing the dosage of drugs.42,43 Nanocarriers can provide protection for their loaded “cargo” and effectively transport them to inaccessible areas, such as the brain.8
![]() |
Figure 2 Various novel nanomaterials for the treatment of neurodegenerative diseases. |
Protein misfolding and aggregation is a common pathogenic hallmark in various neurodegenerative disorders, including AD, PD and HD.2 Studies have shown that nanomaterials can act as bioactive nanomedicines to eliminate reactive oxygen species (ROS) and even suppress toxic protein aggregation.21,44 More importantly, some nanomaterials possess enzymatic properties that can effectively catalyze enzyme substrates under mild conditions.45 They can serve as an alternative to natural enzymes and are expected to provide new ideas and approaches for the treatment of neurodegenerative diseases.20,46 Additionally, nanoparticles can also act as bioactive nanomedicines to clear abnormally aggregated proteins by modulating the autophagy-lysosomal pathway (ALP) and the ubiquitin proteasome system (UPS) pathways.47,48
In addition, nanomaterials can also be used for imaging/contrast/tracing in neurodegenerative disease treatment. Nanomaterials including rare-earth fluorescent materials, metal nanoclusters as well as quantum dots (QDs) can be exploited as fluorescent agents for bioimaging.49,50 Also of note, due to the excellent magnetic properties and optical properties, gold nanoparticles (AuNPs) as well as superparamagnetic iron oxide nanoparticles (SPIONs) can also serve as contrast agents for medical real-time imaging.51,52
Combined Nanotherapeutic and Stem Cell Therapeutic Strategies for the Treatment of Neurodegenerative Diseases
The dimensionality of nanomaterials plays an important role in the formation of their physical, chemical, and biological properties. Nanomaterials can be classified into zero-dimensional (0D, three dimensions are in the range of nanoscale), eg, nanoparticles; one-dimensional (1D, one dimension is not in the nanoscale range), eg, nanotubes, nanowires, and nanofibers; and two-dimensional (2D, two dimensions are not in the nanoscale range), eg, graphene and polymer membranes.53 0D nanomaterials are more suitable as bioactivate supplements, nanocarriers or cell labels for stem cell therapy; 1D or 2D nanomaterials and their composites are more worthy of investigation and exploration as 2D/three-dimensional (3D) nanoscaffolds for stem cell differentiation.
Currently, nanotherapeutic strategies as well as stem cell therapy have shown some advantages and certainly some inadequacies in the treatment of neurodegenerative diseases, respectively. The combination of the two methods could have advantages complementary to each other and improve the therapeutic efficacy. Therefore, stem cell therapy in collaboration with nanotherapeutic strategies will show great potential for the treatment of neurodegenerative diseases (Figure 3).
Nanoparticles as Bioactivate Supplements for Stem Cell Neural Differentiation
In addition to chemical stimuli, physical stimuli can also influence the fate of stem cell differentiation. The size, shape, charge, and surface modification of nanoparticles may all have an impact on the differentiation fate of stem cells.16,54,55 Nanoparticles can act as mechanical stimuli to activate certain signaling pathways in stem cells, thereby inducing differentiation.56 Thus far, nanoparticles including carbon nanoparticles, metal nanoparticles, semiconductor nanoparticles, and DNA nanostructures have been demonstrated to promote stem cells toward neural differentiation through their physicochemical properties.57–61
Carbon nanoparticles, including carbon nanotubes (CNTs), graphene, and carbon 60 (C60), have been studied for neural differentiation of stem cells.55 For example, Ren et al synthesized a water-soluble fullerene C60 derivative by modifying amino acid (alanine) residues on its surface (Ala-C60).62 The results show that Ala-C60 not only promoted the proliferation and differentiation of NSCs into neurons but also could resist oxidative stress.62 In addition, co-transplantation of CNTs/human olfactory bulb-derived NSCs mixture restored cognitive deficits and neurodegenerative changes in a trimethyltin-induced neurodegenerative rat model.63 Here, CNTs appeared to provide support while increasing the tendency of transplanted NSCs to differentiate into neurons rather than glia and thus may serve as promising candidates in stem cell therapy for different neurodegenerative diseases.63
In addition to the general properties that nanomaterials possess, metal nanomaterials have unique optical, electrical, and magnetic properties that can regulate stem cell fate through the introduction of external electromagnetic fields (EMF).64–66 As representative metal nanoparticles, AuNPs are known for their optoelectronic properties, biosafety, and high flexibility in particle size, shape, and functional structures.23,67 AuNPs become transiently magnetized when exposed to a specific EMF frequency, thus enabling more direct transmission of EMF energy to the target cells, which in turn regulates the stem cell fate.65,68 Chang et al injected arginine-glycine-aspartic acid (RGD)-conjugated AuNPs into the mouse hippocampal dentate gyrus simultaneously with EMF stimulation and showed that they could effectively activate adult brain NSCs in the mouse brain and increase hippocampal neurogenesis in the brains of an aged and progeria mouse model, thus alleviating aging symptoms.65 Similarly, electromagnetic AuNPs facilitate efficient direct lineage reprogramming to induce dopamine neurons under specific EMF conditions, effectively and non-invasively alleviating symptoms in a mouse PD model.68
Selenium nanoparticles (SeNPs), as a kind of semiconductor nanomaterial, can scavenge free radicals and are an effective antioxidant with neuroprotective effects.69 In AD rat models, SeNPs not only improve cognitive impairment but also increase the level of BDNF and reduce oxidative stress in the brain.70,71 Co-administration of SeNPs and MSCs may provide a suitable environment for the proliferation and differentiation of transplanted cells, as well as improve cell survival, which is better than that of SeNPs or MSCs treatment alone.70,71
Nanomaterials as Carriers to Deliver Drugs and Stem Cells for Neurodegenerative Disease Treatment
As mentioned above, with the advantages of good biocompatibility and easy functionalization, nanoparticles are ideal carriers for nucleic acid and drug delivery in vitro and in vivo and have great potential for biomedicine applications. These carriers can load and transport cargos by adsorption, embedding, or covalent binding. Various nanomaterials, such as inorganic nanoparticles, polymer nanoparticles, DNA nanostructures, and lipid nanoparticles, have shown great potential in stem cell therapy of neurodegenerative diseases.72–79 Among them, composite nanocarriers, which have multiple functions and can act coordinately and synergistically with each other, have attracted increasing attention. Zhang et al constructed a traceable PCB-based poly (2-hydroxyethyl methacrylate)-RA-poly (carboxybetaine) cell-penetrating peptide (PHEMA-RA-PCB-CPP)/SPIONs/siSOX9 delivery system with temporally controlled release of siSOX9 and RA, which can effectively control the differentiation of NSCs into neurons and significantly improve the cognition and memory of AD mice.80 Meanwhile, due to the presence of SPIONs in this delivery system, it can also be tracked by magnetic resonance imaging (MRI) in vivo. Notably, during stem cell therapy, pathologically high levels of oxidative stress at the injury site can damage transplanted/newborn neurons, thereby affecting the efficacy of stem cell therapy, and nanomaterials with nanozyme activity appear to address this problem well.19,20,81 Ceria-decorated metal-organic framework nanoparticles with nanozyme activity can be used to co-deliver siSOX9 and RA to promote neurogenesis.82 On the one hand, the cooperation of siSOX9 and RA can well promote neuronal directional differentiation. On the other hand, ceria possesses superoxide dismutase (SOD)-like and catalase (CAT)-like activities, which can eliminate ROS and avoid its oxidative damage to newborn neurons, thereby prolonging survival.82 Altogether, these promising results imply that the novel multifunctional nanocomposites are promising drug carriers for the treatment of neurodegenerative diseases.
In addition to being nucleic acid and drug delivery vehicles, nanomaterials also have the ability to promote stem cell migration and even deliver stem cells across the BBB. Studies have shown that, in the 6-Hydroxydopamine hydrobromide (6-OHDA)-induced PD mouse model, after intracerebral injection of dextran-coated iron oxide nanoparticles (Dex-IONPs)-labeled human MSCs (hMSCs) in a 6-OHDA-induced PD mouse model, Dex-IONPs could promote hMSCs migration to the site of DA neuron injury and induce their differentiation into DA-like neurons.83 Moreover, the homing nature of stem cells, especially MSCs, makes it possible to treat neurodegenerative diseases by noninvasive stem cell transplantation. However, in the absence of exogenous carriers, only a few stem cells can enter the brain after transplantation by noninvasive routes. With magnetic targeted cell delivery technology, magnetic labeling of MSCs with SPIONs, accompanied by the application of an external magnet on the region of interest, greatly improves the delivery and homing of stem cells through the BBB into the target tissue in a rat model of PD.84 All in all, while providing protection to their loaded stem cells and homing to the lesion site for the repair of damaged neurons, nanocarriers can also take advantage of their own biological activity or nourish neurons via loaded relevant drugs, eliminate oxidative stress, or even scavenge aberrant aggregated proteins.
Nanomaterials as Scaffolds for Stem Cell Neural Differentiation
2D Extracellular Matrix
2D cell culture in Petri dishes is a traditional cell culture method in vitro. Some physical and biological factors, such as growth factors, hormones, and other chemical or biological molecules, as well as the extracellular matrix, can determine the differentiation fate of stem cells.85,86 Among them, the surface chemistry, stiffness, alignment, topography, and several other parameters of the cell culture matrix (substrate) are important factors in stem cell differentiation.87–89 Combining the unique characteristics of nanomaterials with advanced cell culture methods can effectively promote the differentiation of stem cells to produce specific cell types of the central nervous system, such as functional neurons.88,90,91
The special topography of nanomaterials has been studied as an extracellular matrix (ECM) to control stem cell growth and differentiation into neural cells.92,93 For example, aligned Gly-Tyr-Ile-Gly-Ser-Arg peptide-functionalized PCL nanofibers exhibited accelerated neural differentiation of MSCs.94 Furthermore, biodegradable nanostructured poly (l-lactide-co ε-Caprolactone) (PLCL) elastomeric scaffolds that combine ordered nanopatterned topography with graphene oxide (GO) functionalization can accelerate neural differentiation of mNSCs into mature neurons and glial cells.25 These results illustrate that the ordered nanotopography may better mimic the natural ECM in promoting neural differentiation of stem cells. In addition, biocompatible silica nanozigzags sculpted by a glancing angle deposition were shown to promote the differentiation of neural stem cells into electrophysiologically active neurons in the absence of traditional chemical growth factors.95 Silica nanozigzags exhibit a unique groove-like topography, and their inherent physical characteristics, such as topographical features and stiffness, may play an important role in promoting specific neuronal phenotypic differentiation.95
The unique photothermal and electrical conductivity of nanomaterials can also serve as an extracellular matrix for stem cell differentiation. AuNPs possess good biocompatibility, chemical and physical stability, effective thermal transduction, optical properties, and easy surface functionalization and can be used for photothermal therapy.96 An artificial extracellular matrix made of gold nanocage-coated glass coverslips can promote the differentiation of rat NSCs into neurons, which can be further enhanced by laser-induced thermal stimulation.97 In addition, graphene film, as a 2D nanomaterial, has good conductivity and can be used as a conductive substrate for stem cell differentiation. Guo et al differentiated AMSCs (cultured on graphene film) into functional neurons within 15 days by placing a rotary magnet on top of a culture system without any inducer.17 In this system, the graphene film acts as a wireless electrical signal generator driven by the electromagnetic induction.17
More importantly, nucleic acids and drugs can also be loaded or assembled onto the surface of scaffolds for further modulation of neural differentiation of stem cells. microRNA-222-containing chitosan nanoparticles combined with silk fibroin nanofibrous scaffolds showed enhanced neuronal differentiation of NSCs.98 Moreover, the release of methylene blue (MB) from GO-coated electrospun nanofibrous scaffolds can modulate the function of neural progenitor cells.99 This MB-loaded nanofibrous scaffold could reduce tau phosphorylation and protect NPCs from apoptosis, which may be a versatile treatment for AD and other neurodegenerative diseases.99
3D Nanoscaffolds
Although the classic 2D cell culture can manipulate the fate of stem cells, cells exist in a 3D form in their natural physiological environment within living organisms. 3D cultures can better replicate this state. Compared with the traditional 2D method, the 3D culture model is closer to the natural microenvironment of cells in tissues and organs and represents cell-cell/cell-matrix interactions and cell migration as they are in vivo.100 3D hybrid nanomaterial scaffolds have been demonstrated to be able to mimic the natural microenvironment to promote stem cell proliferation and differentiation for stem cell-based tissue engineering.101,102 Moreover, 3D nanostructured scaffolds can be transplanted into animals and provide support and protection for the stem cells they carry.22
Inorganic and carbon nanomaterials are ideal materials for 3D nanoscaffolds due to their unique biological and physicochemical properties and nanotopography. 3D nanoscaffolds are usually composites composed of some natural polymers (eg, chitosan, collagen, hyaluronic acid (HA), or fibrin) and synthetic nanomaterials (eg, PLGA, PCL, CNTs, or GO).103 Natural source nanomaterials can guarantee biocompatibility for stem cell differentiation due to their good biodegradability and low antigenicity,103,104 while synthetic nanomaterials can be modified in terms of surface topography, mechanical properties, and electrical properties, which make them favorable for the differentiation of stem cells into neural cells.105,106 Although 3D nanoscaffolds have shown great application prospects in nerve regeneration for degenerative diseases, nanocomposites are often restricted due to their nonbiodegradability, poorly controlled release of drugs, and limited biocompatibility, thus delaying their clinical application. To solve these problems, a bioactive 3D reduced graphene oxide (rGO)-collagen hybrid scaffold composed of type I collagen as a basic material and assembled on the surface of a layer of rGO nanosheets was developed.107 This 3D rGO-collagen hybrid scaffold can not only maintain the porous 3D structure and good biocompatibility but also delay the biodegradation rate and endow the scaffold with good conductivity, ultimately promoting MSC proliferation and differentiation into neural cells.107 In addition, Yang et al developed a neurogenic drug DAPT(N-[N-(3,5-difluorophenacetyl)-L-alanyl]-S-phenylglycine t-butyl ester)-loaded 3D-MnO2-laminin hybrid biodegradable nanoscaffold.13 With degradable and controllable drug release, this hybrid nanoscaffold is a useful tool to improve the survival of stem cells and induce neuronal differentiation in vivo; thus, it may be a new means to treat neurodegenerative diseases.13 Furthermore, DNA/histone proteins/K6-polyethylene glycol (PEG)-RGD supramolecular fibers have been fabricated as new 3D scaffolds that provide a biocompatible microenvironment for NSC encapsulation and in situ differentiation into oligodendrocytes, showing great potential in neural differentiation.100 Supramolecular fibers provide new options for novel natural non-toxic scaffolds. They are biomacromolecules formed by the interfacial polyelectrolyte complexation (IPC) process of DNA and histones that can be naturally metabolized into small molecules; various bioactive molecules such as growth factors and chemokines can also be incorporated to further regulate stem cell fate.100 In conclusion, 3D nanoscaffolds are promising candidates for improving cell survival and differentiation in patients with neurodegenerative diseases undergoing stem cell transplantation. Notably, this approach still needs to overcome several major obstacles, including the sudden release of drugs, insufficient cell adhesion support, and slow degradation rate of scaffolds. Researchers are actively developing new nanomaterials, using biocompatible, degradable nanomaterials, and functionalizing their surfaces to address these issues.
Nanoparticle-Based Stem Cell Labeling and Tracking
Stem cell therapy is a promising technique for neurodegenerative disease treatment, but how to monitor the transplanted cells remains a challenge. Monitoring the injected/transplanted cells is required to determine their function and fate, as well as the optimal cell type, route, dose, and timing of administration.108–110 In the past, the success of transplantation was usually assessed by evaluating the improvement of symptoms. Although a few patients have significant improvement in clinical studies, others usually have limited or even no improvement in symptoms. Additionally, limited information is known about the distribution, migration, differentiation, and survival of transplanted stem cells in the brain, which represents a major obstacle to the clinical application of stem cells. Therefore, simple and effective noninvasive cell tracking and imaging technology is urgently needed to evaluate the fate of transplanted stem cells in real time.
Noninvasive monitoring methods can determine the safety and efficacy of cell therapy by tracking the location of transplanted cells in real time, and optimal doses and routes of administration can be selected based on cell distribution; thus, monitoring methods provide critical information for the progress of cell replacement therapy.111,112 The main brain imaging techniques that can be used to track cells in vivo are MRI, X-ray computed tomography (CT), positron emission tomography (PET), and single-photon emission computed tomography (SPECT).113–115 However, these techniques usually require appropriate contrast agents to label transplanted cells to enhance contrast. Nanomaterials are promising candidates for contrast agents because of their unique optical properties, cellular uptake, surface functionalization, and good biocompatibility. Utilizing nanoparticles for cell tracking and imaging typically consists of two steps: (I) labeling cells in vitro using appropriate nanoparticles as contrast agents; and (II) transplantation/injection of labeled cells to the target area, followed by the selection of suitable imaging techniques for noninvasive imaging.
MRI allows long-term tracking of transplanted/injected cells in a noninvasive manner to see if transplantation is successful, with the only requirement being the use of appropriate contrast agents for labeling transplanted cells. Magnetic nanoparticles, especially gadolinium (III) chelates and SPIONs, are the most commonly used contrast agents in MRI detection.52,116 In recent years, SPION-labeled stem cell tracking for MRI has been used in studies on several neurodegenerative diseases, including AD, PD, and HD.84,117–120
To reduce the accumulation and cytotoxicity of SPIONs in tissues and maximize biocompatibility, biopolymers are usually coated on their surface.83 Hour et al labeled Wharton’s jelly-derived MSCs with dextran-coated SPIONs and delivered them magnetically to the hippocampal region of AD rat brains.118 Studies have shown that dextran-coated SPIONs can deliver MSCs to reach the hippocampus and improve AD cognitive impairment by attenuating neuronal degeneration and enhancing cholinergic function in the hippocampus.118 At present, most methods for stem cell labeling are nonspecific and rely on electrostatic interactions between cells and nanoparticles. To solve this problem, Egawa et al constructed a DNA hybridization system for labeling NSCs with SPIONs for MRI monitoring post-transplantation.51 In this hybridization system, oligo[dT]20 ssDNA and lipid molecule DPPE (1,2-dipalmitoyl-sn-glycero-3-phosphoethanolamine) were conjugated by using PEG as a linker (oligo[dT]20-PEG-DPPE, which can be anchored to cell membranes via its hydrophobic DPPE tail), while the complementary ssDNA (oligo[dA]20) was conjugated to maleic acid-coated SPIONs (oligo[dA]20-SPIONs). NSC labeling could then be performed simply by DNA hybridization, which improved the specificity of NSC labeling.51 In the rat striatum, labeled cells can be detected by MRI imaging for up to one month after transplantation.51 In addition, magnetic nanomaterials can also be fabricated into composites with other nanomaterials to improve their biocompatibility, cellular uptake, or thermal stability for better in vivo MR imaging. For example, in vivo tracking of a PD mouse model after stem cell transplantation by MRI revealed that adipose-derived MSC transplantation using magnetic silica core–shell nanoparticles as a contrast agent increased the distribution to the brain, particularly the substantia nigra, which may be a potential therapeutic strategy for PD.116 Meanwhile, DNA-gadolinium-AuNPs exhibited improved T1 contrast and excellent cellular uptake that can be visualized by MRI imaging to better understand the survival and location of implanted cells.52
Several studies have also explored the use of AuNPs for cell labeling and tracking the movement and behavior of cells after transplantation in vivo by CT imaging.23,52 CT imaging is one of the more widely used medical imaging techniques due to its convenience, rapidity, and high spatial resolution.121 Gold provides better contrast with its higher atomic number and X-ray absorption coefficient compared with the clinical contrast agent iodine.122 Therefore, it’s in vivo labeling situation can be more clearly monitored in real time by combining CT imaging techniques. Kim et al developed nanocomplexes for stem cell tracking by coating PLL and rhodamine isothiocyanate B (RITC) on the surface of 40 nm citrate-stabilized AuNPs.121 After transplantation into the rat brain, labeled hMSCs could be clearly visualized using a micro-CT scanner, whereas cell viability, proliferation, and differentiation were not affected.121 Similarly, using this technique, Perets et al traced the migration and homing patterns of exosomes derived from MSCs (MSC-exo) administered intranasally in different brain pathologies (including AD and PD).123 The imaging technique enables noninvasive, longitudinal neuroimaging and whole-brain tracking, which can be used to diagnose brain deficiencies and further facilitate targeted drug delivery.
It is worth noting that there is an inherent question with any form of nanoparticle labeling, that is, whether the observed signal represents live cells during long-term imaging, which seems to be addressed only by the addition of a reporter gene, as it is only expressed by live cells.121 However, the incorporation of reporter genes brings risks of unstable transfection, cell biological changes, immunogenicity, and low sensitivity in vivo.23 To summarize, although there are still some problems to be solved in long-term noninvasive imaging, the greatest value of nanomaterial-based cell imaging is that it can immediately image cell distribution and verify the accuracy of cell delivery.
Conclusions and Future Perspectives
Both stem cell therapy and nanotherapeutic strategies have shown great advantages in the treatment of neurodegenerative diseases. However, each treatment has limitations, and there is currently no therapy that can cure neurodegenerative diseases. Therefore, combining stem cell therapy with nanotherapeutic strategies would greatly enhance the therapeutic effects on neurodegenerative diseases, providing new insights into the treatment of neurodegenerative diseases. The schematic representation of the application of nanomaterials in stem cell therapy of neurodegenerative diseases is shown in Figure 4. The application of various nanomaterials in stem cell therapy for neurodegeneration is mentioned above, and a summary is listed in Table 1.
![]() |
Table 1 A Summary of the Application of Nanomaterials and Stem Cells in Cooperation for the Treatment of Neurodegenerative Diseases |
![]() |
Figure 4 Schematic representation of the application of nanomaterials in stem cell therapy of neurodegenerative diseases. |
Despite many advantages, the clinical application of nanocarriers and nano contrast agents still faces several challenges, such as potential cytotoxicity, batch-to-batch variation of polymeric nanoparticles, and nonbiodegradability of inorganic nanoparticles.124,125 Therefore, it is necessary to improve the synthesis method and develop multifunctional, biocompatible, and degradable composite nanocarriers. In addition, to improve the controlled release and targeting of drugs of nanocarriers, novel nanomaterials with appropriate surface modification and specific physical and biochemical characteristics should be further developed.
Nanotherapeutic strategies combined with stem cell therapy, from laboratory experiments to clinical application, are still in the process of continuous improvement and development. The safety profile of nanotherapeutic strategies, as well as how they improve the efficacy of stem cells in treating neurodegenerative diseases, is also a research hotspot, and biocompatible degradable materials would be the first choice.
In conclusion, although various obstacles and challenges remain, nanotherapeutic strategies combined with stem cell therapy remain a promising approach for the future treatment of neurodegenerative diseases. It is believed that, as research continues to deepen and a variety of new nanomaterials are discovered, people will surely benefit from this technology.
Author Contributions
All authors have made major contributions to this work; participated in drafting, revising, and critically reviewing the article; gave final approval of the version to be published; have agreed on the journal to which the article has been submitted; and agree to be accountable for all aspects of the work.
Funding
This work was supported by grants from the National Natural Science Foundation of China (81771521), and Project of Natural Science Foundation of Liaoning Province (2019-ZD-0943).
Disclosure
The authors declare no conflict of interest.
References
1. Heemels MT. Neurodegenerative diseases. Nature. 2016;539(7628):179. doi:10.1038/539179a
2. Vaquer-Alicea J, Diamond MI. Propagation of protein aggregation in neurodegenerative diseases. Annu Rev Biochem. 2019;88:785–810. doi:10.1146/annurev-biochem-061516-045049
3. Karabiyik CFR, Park S, Pavel M, Rubinsztein D. Autophagy in ageing and ageing-related neurodegenerative diseases. Ageing Neur Dis. 2021;1(1):2.
4. Dugger BN, Dickson DW. Pathology of neurodegenerative diseases. Cold Spring Harb Perspect Biol. 2017;9(7):a028035. doi:10.1101/cshperspect.a028035
5. Sivandzade F, Cucullo L. Regenerative stem cell therapy for neurodegenerative diseases: an overview. Int J Mol Sci. 2021;22(4). doi:10.3390/ijms22042153
6. Furtado D, Bjornmalm M, Ayton S, Bush AI, Kempe K, Caruso F. Overcoming the blood-brain barrier: the role of nanomaterials in treating neurological diseases. Adv Mater. 2018;30(46):e1801362. doi:10.1002/adma.201801362
7. Chen J, Yuan M, Madison CA, Eitan S, Wang Y. Blood-brain barrier crossing using magnetic stimulated nanoparticles. J Control Release. 2022;345:557–571. doi:10.1016/j.jconrel.2022.03.007
8. Saraiva C, Praca C, Ferreira R, Santos T, Ferreira L, Bernardino L. Nanoparticle-mediated brain drug delivery: overcoming blood-brain barrier to treat neurodegenerative diseases. J Control Release. 2016;235:34–47. doi:10.1016/j.jconrel.2016.05.044
9. Kumar A, Narayanan K, Chaudhary RK, et al. Current Perspective of Stem Cell Therapy in Neurodegenerative and Metabolic Diseases. Mol Neurobiol. 2017;54(9):7276–7296. doi:10.1007/s12035-016-0217-4
10. Martinez B, Peplow PV. Biomaterial and tissue-engineering strategies for the treatment of brain neurodegeneration. Neural Regen Res. 2022;17(10):2108–2116. doi:10.4103/1673-5374.336132
11. Joyce N, Annett G, Wirthlin L, Olson S, Bauer G, Nolta JA. Mesenchymal stem cells for the treatment of neurodegenerative disease. Regen Med. 2010;5(6):933–946. doi:10.2217/rme.10.72
12. De Gioia R, Biella F, Citterio G, et al. Neural stem cell transplantation for neurodegenerative diseases. Int J Mol Sci. 2020;21(9):3103. doi:10.3390/ijms21093103
13. Yang L, Chueng SD, Li Y, et al. A biodegradable hybrid inorganic nanoscaffold for advanced stem cell therapy. Nat Commun. 2018;9(1):3147. doi:10.1038/s41467-018-05599-2
14. Yamanaka S. Pluripotent stem cell-based cell therapy-promise and challenges. Cell Stem Cell. 2020;27(4):523–531. doi:10.1016/j.stem.2020.09.014
15. Vissers C, Ming GL, Song H. Nanoparticle technology and stem cell therapy team up against neurodegenerative disorders. Adv Drug Deliv Rev. 2019;148:239–251. doi:10.1016/j.addr.2019.02.007
16. Wei M, Li S, Le W. Nanomaterials modulate stem cell differentiation: biological interaction and underlying mechanisms. J Nanobiotechnology. 2017;15(1):75. doi:10.1186/s12951-017-0310-5
17. Guo Z, Sun C, Yang H, et al. Regulation of neural differentiation of ADMSCs using graphene-mediated wireless-localized electrical signals driven by electromagnetic induction. Adv Sci. 2022;9(14):e2104424. doi:10.1002/advs.202104424
18. Zhong H, Wu YX, Yu S, et al. Two-photon CQDs-based dual-mode nanoprobe for fluorescence imaging and magnetic resonance imaging of intracellular wide pH. Anal Chem. 2021;93(14):5691–5699. doi:10.1021/acs.analchem.0c04605
19. Adhikari A, Mondal S, Das M, et al. Incorporation of a biocompatible nanozyme in cellular antioxidant enzyme cascade reverses huntington’s like disorder in preclinical model. Adv Healthc Mater. 2021;10(7):e2001736. doi:10.1002/adhm.202001736
20. Singh N, Savanur MA, Srivastava S, D’silva P, Mugesh G. A redox modulatory Mn3 O4 nanozyme with multi-enzyme activity provides efficient cytoprotection to human cells in a Parkinson’s disease model. Angew Chem Int Ed Engl. 2017;56(45):14267–14271. doi:10.1002/anie.201708573
21. Debnath K, Pradhan N, Singh BK, Jana NR, Jana NR. Poly(trehalose) nanoparticles prevent amyloid aggregation and suppress polyglutamine aggregation in a huntington’s disease model mouse. ACS Appl Mater Interfaces. 2017;9(28):24126–24139. doi:10.1021/acsami.7b06510
22. Qiao S, Liu Y, Han F, et al. An intelligent neural stem cell delivery system for neurodegenerative diseases treatment. Adv Healthc Mater. 2018;7(12):e1800080. doi:10.1002/adhm.201800080
23. Chandrasekaran R, Madheswaran T, Tharmalingam N, Bose RJ, Park H, Ha DH. Labeling and tracking cells with gold nanoparticles. Drug Discov Today. 2021;26(1):94–105. doi:10.1016/j.drudis.2020.10.020
24. Li W, Qiu J, Li XL, et al. BBB pathophysiology-independent delivery of siRNA in traumatic brain injury. Sci Adv. 2021;7(1):eabd6889.
25. Polo Y, Luzuriaga J, Iturri J, et al. Nanostructured scaffolds based on bioresorbable polymers and graphene oxide induce the aligned migration and accelerate the neuronal differentiation of neural stem cells. Nanomedicine. 2021;31:102314. doi:10.1016/j.nano.2020.102314
26. Parmar M, Grealish S, Henchcliffe C. The future of stem cell therapies for Parkinson disease. Nat Rev Neurosci. 2020;21(2):103–115. doi:10.1038/s41583-019-0257-7
27. Parmar M. Towards stem cell based therapies for Parkinson’s disease. Development. 2018;145(1). doi:10.1242/dev.156117
28. Chan HJ, Yanshree RJ, Tipoe GL, Fung ML, Lim LW, Lim LW. Therapeutic potential of human stem cell implantation in alzheimer’s disease. Int J Mol Sci. 2021;22(18):10151. doi:10.3390/ijms221810151
29. Wang Y, Ji X, Leak RK, Chen F, Cao G. Stem cell therapies in age-related neurodegenerative diseases and stroke. Ageing Res Rev. 2017;34:39–50. doi:10.1016/j.arr.2016.11.002
30. Cone AS, Yuan X, Sun L, et al. Mesenchymal stem cell-derived extracellular vesicles ameliorate Alzheimer’s disease-like phenotypes in a preclinical mouse model. Theranostics. 2021;11(17):8129–8142. doi:10.7150/thno.62069
31. Hattiangady B, Kuruba R, Shuai B, Grier R, Shetty AK. Hippocampal neural stem cell grafting after status epilepticus alleviates chronic epilepsy and abnormal plasticity, and maintains better memory and mood function. Aging Dis. 2020;11(6):1374–1394. doi:10.14336/AD.2020.1020
32. Fayazi N, Sheykhhasan M, Soleimani Asl S, Najafi R. Stem cell-derived exosomes: a new strategy of neurodegenerative disease treatment. Mol Neurobiol. 2021;58(7):3494–3514. doi:10.1007/s12035-021-02324-x
33. Sherman LS, Romagano MP, Williams SF, Rameshwar P. Mesenchymal stem cell therapies in brain disease. Semin Cell Dev Biol. 2019;95:111–119. doi:10.1016/j.semcdb.2019.03.003
34. Staff NP, Jones DT, Singer W. Mesenchymal stromal cell therapies for neurodegenerative diseases. Mayo Clin Proc. 2019;94(5):892–905. doi:10.1016/j.mayocp.2019.01.001
35. Andrzejewska A, Dabrowska S, Lukomska B, Janowski M. Mesenchymal stem cells for neurological disorders. Adv Sci. 2021;8(7):2002944. doi:10.1002/advs.202002944
36. Gonzalez R, Hamblin MH, Lee JP. Neural stem cell transplantation and CNS diseases. CNS Neurol Disord Drug Targets. 2016;15(8):881–886. doi:10.2174/1871527315666160815164247
37. Ford E, Pearlman J, Ruan T, et al. Human pluripotent stem cells-based therapies for neurodegenerative diseases: current status and challenges. Cells. 2020;9(11):2517. doi:10.3390/cells9112517
38. Abdi S, Javanmehr N, Ghasemi-Kasman M, Bali HY, Pirzadeh M. Stem cell-based therapeutic and diagnostic approaches in alzheimer’s disease. Curr Neuropharmacol. 2021;20(6):1093–1115.
39. Carradori D, Eyer J, Saulnier P, Preat V, Des Rieux A. The therapeutic contribution of nanomedicine to treat neurodegenerative diseases via neural stem cell differentiation. Biomaterials. 2017;123:77–91. doi:10.1016/j.biomaterials.2017.01.032
40. Nguyen TT, Dung Nguyen TT, Vo TK, et al. Nanotechnology-based drug delivery for central nervous system disorders. Biomed Pharmacother. 2021;143:112117. doi:10.1016/j.biopha.2021.112117
41. Tosi G, Vandelli MA, Forni F, Ruozi B. Nanomedicine and neurodegenerative disorders: so close yet so far. Expert Opin Drug Deliv. 2015;12(7):1041–1044. doi:10.1517/17425247.2015.1041374
42. Rezaei A, Rafieian F, Akbari-Alavijeh S, Kharazmi MS, Jafari SM. Release of bioactive compounds from delivery systems by stimuli-responsive approaches; triggering factors, mechanisms, and applications. Adv Colloid Interface Sci. 2022;307:102728. doi:10.1016/j.cis.2022.102728
43. Cardoso BD, Cardoso VF, Lanceros-Mendez S, Castanheira EMS. Solid magnetoliposomes as multi-stimuli-responsive systems for controlled release of doxorubicin: assessment of lipid formulations. Biomedicines. 2022;10(5):1207. doi:10.3390/biomedicines10051207
44. Decoteau W, Heckman KL, Estevez AY, et al. Cerium oxide nanoparticles with antioxidant properties ameliorate strength and prolong life in mouse model of amyotrophic lateral sclerosis. Nanomedicine. 2016;12(8):2311–2320. doi:10.1016/j.nano.2016.06.009
45. Cormode DP, Gao L, Koo H. Emerging biomedical applications of enzyme-like catalytic nanomaterials. Trends Biotechnol. 2018;36(1):15–29. doi:10.1016/j.tibtech.2017.09.006
46. Wei M, Lee J, Xia F, et al. Chemical design of nanozymes for biomedical applications. Acta Biomater. 2021;126:15–30. doi:10.1016/j.actbio.2021.02.036
47. Zhang L, Wei PF, Song YH, et al. MnFe2O4 nanoparticles accelerate the clearance of mutant huntingtin selectively through ubiquitin-proteasome system. Biomaterials. 2019;216:119248. doi:10.1016/j.biomaterials.2019.119248
48. Arotcarena ML, Soria FN, Cunha A, et al. Acidic nanoparticles protect against alpha-synuclein-induced neurodegeneration through the restoration of lysosomal function. Aging Cell. 2022;21(4):e13584. doi:10.1111/acel.13584
49. Xu Q, Gao J, Wang S, Wang Y, Liu D, Wang J. Quantum dots in cell imaging and their safety issues. J Mater Chem B. 2021;9(29):5765–5779. doi:10.1039/D1TB00729G
50. Labrador-Paez L, Ximendes EC, Rodriguez-Sevilla P, et al. Core-shell rare-earth-doped nanostructures in biomedicine. Nanoscale. 2018;10(27):12935–12956. doi:10.1039/C8NR02307G
51. Egawa EY, Kitamura N, Nakai R, Arima Y, Iwata H. A DNA hybridization system for labeling of neural stem cells with SPIO nanoparticles for MRI monitoring post-transplantation. Biomaterials. 2015;54:158–167. doi:10.1016/j.biomaterials.2015.03.017
52. Nicholls FJ, Rotz MW, Ghuman H, Macrenaris KW, Meade TJ, Modo M. DNA-gadolinium-gold nanoparticles for in vivo T1 MR imaging of transplanted human neural stem cells. Biomaterials. 2016;77:291–306. doi:10.1016/j.biomaterials.2015.11.021
53. Zhang C, Xie B, Zou Y, et al. Zero-dimensional, one-dimensional, two-dimensional and three-dimensional biomaterials for cell fate regulation. Adv Drug Deliv Rev. 2018;132:33–56. doi:10.1016/j.addr.2018.06.020
54. Hao M, Zhang Z, Liu C, et al. Hydroxyapatite nanorods function as safe and effective growth factors regulating neural differentiation and neuron development. Adv Mater. 2021;33(33):e2100895. doi:10.1002/adma.202100895
55. Xia L, Zhu W, Wang Y, He S, Chai R. Regulation of neural stem cell proliferation and differentiation by graphene-based biomaterials. Neural Plast. 2019;2019:3608386. doi:10.1155/2019/3608386
56. Lee JM, Kang WS, Lee KG, et al. Combinatorial biophysical cue sensor array for controlling neural stem cell fate. Biosens Bioelectron. 2020;156:112125. doi:10.1016/j.bios.2020.112125
57. Wei M, Li S, Yang Z, Zheng W, Le W. Gold nanoparticles enhance the differentiation of embryonic stem cells into dopaminergic neurons via mTOR/p70S6K pathway. Nanomedicine. 2017;12(11):1305–1317. doi:10.2217/nnm-2017-0001
58. Ma W, Xie X, Shao X, et al. Tetrahedral DNA nanostructures facilitate neural stem cell migration via activating RHOA/ROCK2 signalling pathway. Cell Prolif. 2018;51(6):e12503. doi:10.1111/cpr.12503
59. Park SJ, Kim S, Kim SY, et al. Highly efficient and rapid neural differentiation of mouse embryonic stem cells based on retinoic acid encapsulated porous nanoparticle. ACS Appl Mater Interfaces. 2017;9(40):34634–34640. doi:10.1021/acsami.7b09760
60. Yang D, Li T, Xu M, et al. Graphene oxide promotes the differentiation of mouse embryonic stem cells to dopamine neurons. Nanomedicine. 2014;9(16):2445–2455. doi:10.2217/nnm.13.197
61. Hung HS, Yang YC, Chang CH, et al. Neural differentiation potential of mesenchymal stem cells enhanced by biocompatible chitosan-gold nanocomposites. Cells. 2022;11(12):1861. doi:10.3390/cells11121861
62. Ren H, Li J, Peng A, et al. Water-soluble, alanine-modified fullerene C60 promotes the proliferation and neuronal differentiation of neural stem cells. Int J Mol Sci. 2022;23(10):5714. doi:10.3390/ijms23105714
63. Marei HE, Elnegiry AA, Zaghloul A, et al. Nanotubes impregnated human olfactory bulb neural stem cells promote neuronal differentiation in Trimethyltin-induced neurodegeneration rat model. J Cell Physiol. 2017;232(12):3586–3597. doi:10.1002/jcp.25826
64. Semeano AT, Tofoli FA, Correa-Velloso JC, et al. Effects of magnetite nanoparticles and static magnetic field on neural differentiation of pluripotent stem cells. Stem Cell Rev Rep. 2022;18(4):1337–1354. doi:10.1007/s12015-022-10332-0
65. Chang Y, Cho B, Lee E, et al. Electromagnetized gold nanoparticles improve neurogenesis and cognition in the aged brain. Biomaterials. 2021;278:121157. doi:10.1016/j.biomaterials.2021.121157
66. Zhang S, Hao M, Gao W, et al. Neuron-like cell differentiation of hADSCs promoted by a copper sulfide nanostructure mediated plasmonic effect driven by near-infrared light. Nanoscale. 2020;12(17):9833–9841. doi:10.1039/D0NR02319A
67. Qu A, Sun M, Kim JY, et al. Stimulation of neural stem cell differentiation by circularly polarized light transduced by chiral nanoassemblies. Nat Biomed Eng. 2021;5(1):103–113. doi:10.1038/s41551-020-00634-4
68. Yoo J, Lee E, Kim HY, et al. Electromagnetized gold nanoparticles mediate direct lineage reprogramming into induced dopamine neurons in vivo for Parkinson’s disease therapy. Nat Nanotechnol. 2017;12(10):1006–1014. doi:10.1038/nnano.2017.133
69. Cong W, Bai R, Li YF, Wang L, Chen C. Selenium nanoparticles as an efficient nanomedicine for the therapy of huntington’s disease. ACS Appl Mater Interfaces. 2019;11(38):34725–34735. doi:10.1021/acsami.9b12319
70. Gholamigeravand B, Shahidi S, Afshar S, et al. Synergistic effects of adipose-derived mesenchymal stem cells and selenium nanoparticles on streptozotocin-induced memory impairment in the rat. Life Sci. 2021;272:119246. doi:10.1016/j.lfs.2021.119246
71. Soleimani Asl S, Amiri I, Samzadeh-Kermani A, Abbasalipourkabir R, Gholamigeravand B, Shahidi S. Chitosan-coated Selenium nanoparticles enhance the efficiency of stem cells in the neuroprotection of streptozotocin-induced neurotoxicity in male rats. Int J Biochem Cell Biol. 2021;141:106089. doi:10.1016/j.biocel.2021.106089
72. Zhao X, Glass Z, Chen J, Yang L, Kaplan DL, Xu Q. mRNA delivery using bioreducible lipidoid nanoparticles facilitates neural differentiation of human mesenchymal stem cells. Adv Healthc Mater. 2021;10(4):e2000938. doi:10.1002/adhm.202000938
73. Kuo YC, Shih-Huang CY, Rajesh R. Enhanced integrin affinity and neural differentiation of induced pluripotent stem cells using Ln5-P4-grafted amphiphilic solid lipid nanoparticles. Mater Sci Eng C Mater Biol Appl. 2021;118:111339. doi:10.1016/j.msec.2020.111339
74. Wu D, Zhang Y, Xu X, et al. RGD/TAT-functionalized chitosan-graft-PEI-PEG gene nanovector for sustained delivery of NT-3 for potential application in neural regeneration. Acta Biomater. 2018;72:266–277. doi:10.1016/j.actbio.2018.03.030
75. Marcuzzo S, Isaia D, Bonanno S, et al. FM19G11-loaded gold nanoparticles enhance the proliferation and self-renewal of ependymal stem progenitor cells derived from ALS mice. Cells. 2019;8(3):279. doi:10.3390/cells8030279
76. Ma W, Shao X, Zhao D, et al. Self-assembled tetrahedral DNA nanostructures promote neural stem cell proliferation and neuronal differentiation. ACS Appl Mater Interfaces. 2018;10(9):7892–7900. doi:10.1021/acsami.8b00833
77. Ferreira R, Fonseca MC, Santos T, et al. Retinoic acid-loaded polymeric nanoparticles enhance vascular regulation of neural stem cell survival and differentiation after ischaemia. Nanoscale. 2016;8(15):8126–8137. doi:10.1039/C5NR09077F
78. Zhang R, Wang Z, Yang Z, et al. RNA-silencing nanoprobes for effective activation and dynamic imaging of neural stem cell differentiation. Theranostics. 2019;9(18):5386–5395. doi:10.7150/thno.35032
79. Wei M, Li S, Yang Z, Cheng C, Li T, Le W. Tetrahedral DNA nanostructures functionalized by multivalent microRNA132 antisense oligonucleotides promote the differentiation of mouse embryonic stem cells into dopaminergic neurons. Nanomedicine. 2021;34:102375. doi:10.1016/j.nano.2021.102375
80. Zhang R, Li Y, Hu B, Lu Z, Zhang J, Zhang X. Traceable nanoparticle delivery of small interfering RNA and retinoic acid with temporally release ability to control neural stem cell differentiation for Alzheimer’s disease therapy. Adv Mater. 2016;28(30):6345–6352. doi:10.1002/adma.201600554
81. Xu Z, Qu A, Wang W, et al. Facet-dependent biodegradable Mn3 O4 nanoparticles for ameliorating Parkinson’s disease. Adv Healthc Mater. 2021;10(23):e2101316. doi:10.1002/adhm.202101316
82. Yu D, Ma M, Liu Z, et al. MOF-encapsulated nanozyme enhanced siRNA combo: control neural stem cell differentiation and ameliorate cognitive impairments in Alzheimer’s disease model. Biomaterials. 2020;255:120160. doi:10.1016/j.biomaterials.2020.120160
83. Chung TH, Hsu SC, Wu SH, et al. Dextran-coated iron oxide nanoparticle-improved therapeutic effects of human mesenchymal stem cells in a mouse model of Parkinson’s disease. Nanoscale. 2018;10(6):2998–3007. doi:10.1039/C7NR06976F
84. Moayeri A, Darvishi M, Amraei M. Homing of Super Paramagnetic Iron Oxide Nanoparticles (SPIONs) labeled adipose-derived stem cells by magnetic attraction in a rat model of Parkinson’s disease. Int J Nanomed. 2020;15:1297–1308. doi:10.2147/IJN.S238266
85. Discher DE, Mooney DJ, Zandstra PW. Growth factors, matrices, and forces combine and control stem cells. Science. 2009;324(5935):1673–1677. doi:10.1126/science.1171643
86. Faissner A, Reinhard J. The extracellular matrix compartment of neural stem and glial progenitor cells. Glia. 2015;63(8):1330–1349. doi:10.1002/glia.22839
87. Yang L, Lee JH, Rathnam C, Hou Y, Choi JW, Lee KB. Dual-enhanced raman scattering-based characterization of stem cell differentiation using graphene-plasmonic hybrid nanoarray. Nano Lett. 2019;19(11):8138–8148. doi:10.1021/acs.nanolett.9b03402
88. Asheghali D, Lee SJ, Furchner A, et al. Enhanced neuronal differentiation of neural stem cells with mechanically enhanced touch-spun nanofibrous scaffolds. Nanomedicine. 2020;24:102152. doi:10.1016/j.nano.2020.102152
89. Lu X, Sun C, Chen L, et al. Stemness maintenance and massproduction of neural stem cells on Poly L-Lactic acid nanofibrous membrane based on piezoelectriceffect. Small. 2022;18(13):e2107236. doi:10.1002/smll.202107236
90. Rawat S, Jain KG, Gupta D, et al. Graphene nanofiber composites for enhanced neuronal differentiation of human mesenchymal stem cells. Nanomedicine. 2021;16(22):1963–1982. doi:10.2217/nnm-2021-0121
91. Rahimzadegan M, Mohammadi Q, Shafieian M, Sabzevari O, Hassannejad Z. Influence of reducing agents on in situ synthesis of gold nanoparticles and scaffold conductivity with emphasis on neural differentiation. Mater Sci Eng C Mater Biol Appl. 2021;134:112634.
92. Hsieh FY, Shrestha LK, Ariga K, Hsu SH. Neural differentiation on aligned fullerene C60 nanowhiskers. Chem Commun. 2017;53(80):11024–11027. doi:10.1039/C7CC06395D
93. Schulte C, Rodighiero S, Cappelluti MA, et al. Conversion of nanoscale topographical information of cluster-assembled zirconia surfaces into mechanotransductive events promotes neuronal differentiation. J Nanobiotechnol. 2016;14:18. doi:10.1186/s12951-016-0171-3
94. Silantyeva EA, Nasir W, Carpenter J, Manahan O, Becker ML, Willits RK. Accelerated neural differentiation of mouse embryonic stem cells on aligned GYIGSR-functionalized nanofibers. Acta Biomater. 2018;75:129–139. doi:10.1016/j.actbio.2018.05.052
95. Zhang S, Sun P, Lin K, et al. Extracellular nanomatrix-induced self-organization of neural stem cells into miniature substantia nigra-like structures with therapeutic effects on parkinsonian rats. Adv Sci. 2019;6(24):1901822. doi:10.1002/advs.201901822
96. Dai X, Zhao X, Liu Y, et al. Controlled synthesis and surface engineering of janus chitosan-gold nanoparticles for photoacoustic imaging-guided synergistic gene/photothermal therapy. Small. 2021;17(11):e2006004. doi:10.1002/smll.202006004
97. Jung S, Harris N, Niyonshuti II, et al. Photothermal response induced by nanocage-coated artificial extracellular matrix promotes neural stem cell differentiation. Nanomaterials. 2021;11(5):1216. doi:10.3390/nano11051216
98. Li Z, Meng Z, Zhao Z. Silk fibroin nanofibrous scaffolds incorporated with microRNA-222 loaded chitosan nanoparticles for enhanced neuronal differentiation of neural stem cells. Carbohydr Polym. 2022;277:118791. doi:10.1016/j.carbpol.2021.118791
99. Wang L, Liu X, Fu J, et al. Release of methylene blue from graphene oxide-coated electrospun nanofibrous scaffolds to modulate functions of neural progenitor cells. Acta Biomater. 2019;88:346–356. doi:10.1016/j.actbio.2019.02.036
100. Zhao H, Xu J, Peng K, et al. Supramolecular nanofibers for encapsulation and in situ differentiation of neural stem cells. Adv Healthc Mater. 2020;9(1):e1901295. doi:10.1002/adhm.201901295
101. Mabrouk M, Ismail E, Beherei H, et al. Biocompatibility of hydroxyethyl cellulose/glycine/RuO2 composite scaffolds for neural-like cells. Int J Biol Macromol. 2022;209(Pt B):2097–2108. doi:10.1016/j.ijbiomac.2022.04.190
102. Ranjan VD, Qiu L, Lee JW, et al. A microfiber scaffold-based 3D in vitro human neuronal culture model of Alzheimer’s disease. Biomater Sci. 2020;8(17):4861–4874. doi:10.1039/D0BM00833H
103. Chen X, Wang Y, Zhou G, Hu X, Han S, Gao J. The combination of nanoscaffolds and stem cell transplantation: paving a promising road for spinal cord injury regeneration. Biomed Pharmacother. 2021;143:112233. doi:10.1016/j.biopha.2021.112233
104. Qu W, Chen B, Shu W, et al. Polymer-based scaffold strategies for spinal cord repair and regeneration. Front Bioeng Biotechnol. 2020;8:590549. doi:10.3389/fbioe.2020.590549
105. Ma Q, Yang L, Jiang Z, et al. Three-dimensional stiff graphene scaffold on neural stem cells behavior. ACS Appl Mater Interfaces. 2016;8(50):34227–34233. doi:10.1021/acsami.6b12305
106. Kim GJ, Lee KJ, Choi JW, An JH. Modified industrial three-dimensional polylactic acid scaffold cell chip promotes the proliferation and differentiation of human neural stem cells. Int J Mol Sci. 2022;23(4):2204.
107. Guo W, Wang S, Yu X, et al. Construction of a 3D rGO-collagen hybrid scaffold for enhancement of the neural differentiation of mesenchymal stem cells. Nanoscale. 2016;8(4):1897–1904. doi:10.1039/C5NR06602F
108. Aarntzen EH, Srinivas M, Punt CJ, Figdor CG, Oyen WJ, De Vries IJ. Insight into the dynamics, localization and magnitude of antigen-specific immune responses by [(18)F]FLT PET imaging. Oncoimmunology. 2012;1(5):744–745. doi:10.4161/onci.19533
109. Bagno L, Hatzistergos KE, Balkan W, Hare JM. Mesenchymal stem cell-based therapy for cardiovascular disease: progress and challenges. Mol Ther. 2018;26(7):1610–1623. doi:10.1016/j.ymthe.2018.05.009
110. Kim J, Chhour P, Hsu J, et al. Use of nanoparticle contrast agents for cell tracking with computed tomography. Bioconjug Chem. 2017;28(6):1581–1597. doi:10.1021/acs.bioconjchem.7b00194
111. Zhang B, Yan W, Zhu Y, et al. Nanomaterials in neural-stem-cell-mediated regenerative medicine: imaging and treatment of neurological diseases. Adv Mater. 2018;30(17):e1705694. doi:10.1002/adma.201705694
112. Yim MS, Hwang YS, Bang JK, et al. Morphologically homogeneous, pH-responsive gold nanoparticles for non-invasive imaging of HeLa cancer. Nanomedicine. 2021;34:102394. doi:10.1016/j.nano.2021.102394
113. Matsuda H. MRI morphometry in Alzheimer’s disease. Ageing Res Rev. 2016;30:17–24. doi:10.1016/j.arr.2016.01.003
114. Johnson KA, Fox NC, Sperling RA, Klunk WE. Brain imaging in Alzheimer disease. Cold Spring Harb Perspect Med. 2012;2(4):a006213. doi:10.1101/cshperspect.a006213
115. Gao T, Wang P, Gong T, et al. Reporter genes for brain imaging using MRI, SPECT and PET. Int J Mol Sci. 2022;23(15):8443.
116. Kim KY, Chang KA. Therapeutic potential of magnetic nanoparticle-based human adipose-derived stem cells in a mouse model of Parkinson’s disease. Int J Mol Sci. 2021;22(2):654.
117. Islam J, So KH, Kc E, et al. Transplantation of human embryonic stem cells alleviates motor dysfunction in AAV2-Htt171-82Q transfected rat model of Huntington’s disease. Stem Cell Res Ther. 2021;12(1):585. doi:10.1186/s13287-021-02653-7
118. Hour FQ, Moghadam AJ, Shakeri-Zadeh A, Bakhtiyari M, Shabani R, Mehdizadeh M. Magnetic targeted delivery of the SPIONs-labeled mesenchymal stem cells derived from human Wharton’s jelly in Alzheimer’s rat models. J Control Release. 2020;321:430–441. doi:10.1016/j.jconrel.2020.02.035
119. Wang Y, Jiang J, Fu X, et al. [email protected] nanoparticle-loaded human umbilical cord mesenchymal stem cells improve the cognitive function in Alzheimer’s disease mice by promoting hippocampal neurogenesis. Nanomedicine. 2022;40:102507. doi:10.1016/j.nano.2021.102507
120. Li Y, Li Y, Ji W, et al. Positively charged polyprodrug amphiphiles with enhanced drug loading and reactive oxygen species-responsive release ability for traceable synergistic therapy. J Am Chem Soc. 2018;140(11):4164–4171. doi:10.1021/jacs.8b01641
121. Kim T, Lee N, Arifin DR, et al. In vivo Micro-CT imaging of human mesenchymal stem cells labeled with Gold-Poly-L-Lysine nanocomplexes. Adv Funct Mater. 2017;27(3). doi:10.1002/adfm.201604213
122. Cole LE, Ross RD, Tilley JM, Vargo-Gogola T, Roeder RK. Gold nanoparticles as contrast agents in x-ray imaging and computed tomography. Nanomedicine. 2015;10(2):321–341. doi:10.2217/nnm.14.171
123. Perets N, Betzer O, Shapira R, et al. Golden exosomes selectively target brain pathologies in neurodegenerative and neurodevelopmental disorders. Nano Lett. 2019;19(6):3422–3431. doi:10.1021/acs.nanolett.8b04148
124. Dippold D, Cai A, Hardt M, et al. Investigation of the batch-to-batch inconsistencies of Collagen in PCL-Collagen nanofibers. Mater Sci Eng C Mater Biol Appl. 2019;95:217–225. doi:10.1016/j.msec.2018.10.057
125. Wang X, Zhong X, Li J, Liu Z, Cheng L. Inorganic nanomaterials with rapid clearance for biomedical applications. Chem Soc Rev. 2021;50(15):8669–8742. doi:10.1039/d0cs00461h
126. Jang SE, Qiu L, Cai X, et al. Aggregation-induced emission (AIE) nanoparticles labeled human embryonic stem cells (hESCs)-derived neurons for transplantation. Biomaterials. 2021;271:120747. doi:10.1016/j.biomaterials.2021.120747
127. Huang D, Cao Y, Yang X, et al. A nanoformulation-mediated multifunctional stem cell therapy with improved beta-amyloid clearance and neural regeneration for Alzheimer’s disease. Adv Mater. 2021;33(13):e2006357. doi:10.1002/adma.202006357