The Past, Present and Future of Spatial Biology
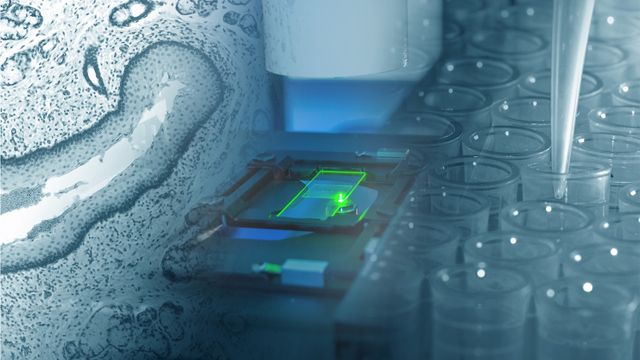
Spatial biology is transforming biomedical research by revealing how cells, molecules and tissues interact in their native environments. This discipline opens a new frontier for understanding complex biological systems, leading to breakthroughs in disease diagnosis, drug development and personalized medicine. However, as the field grows, challenges in data integration and analysis are surfacing, demanding robust technological advancements.
This listicle uncovers spatial biology’s journey, from foundational techniques to cutting-edge technologies that define the present.
Download this listicle to explore:
- Key developments that have shaped spatial biology
- Applications of spatial biology in cancer research, neurodegenerative diseases and drug discovery
- The use of emerging technologies to overcome challenges in data complexity
Listicle
1
The Past, Present and Future of
Spatial Biology
Bree Foster, PhD
The phrase “nothing exists in a vacuum” applies to almost every aspect of life, including the very cells
that make up our bodies. Just as human behavior cannot be fully understood in isolation from external
forces; cells, tissues and organs must be studied within their natural, dynamic surroundings to truly
understand their functions. Cells are constantly interacting with their environment, responding to external
stimuli, communicating with neighboring cells and reacting to distant signals within the body.
This is precisely what spatial biology explores, adding a new dimension to the study of life by examining
how molecules, cells and tissues are organized and interact within their natural environments.
Spatial biology, encompassing fields like spatial transcriptomics and spatial proteomics, allows
researchers to capture the complexity of living systems, leading to more accurate diagnostics and
personalized treatments.1
Recognized by Nature Methods as the “method of the year” in 2021, spatial omics has the potential to
transform disease research, clinical medicine and drug discovery.2 This cutting-edge approach enables
the identification of disease biomarkers, drug resistance mechanisms and the optimization of treatment
strategies.3,4 For example, researchers have discovered that certain cancer patients have unique
spatial patterns of cell communication that make them resistant to conventional therapies.5,6 By blocking
these communication pathways through immunotherapy, the effectiveness of standard treatments
can be significantly enhanced.5
While spatial transcriptomics and proteomics methods are quickly becoming standard practice, researchers
have been trying to understand cellular function in a true morphological context for a long
time. In this listicle, we explore the key milestones of spatial profiling methods from their inception to
advances in resolution and multiplex detection.
Immunohistochemistry and in situ hybridization
Conducting spatial molecular measurements on tissue sections has a long history. Techniques such
as immunohistochemistry (IHC) and in situ hybridization (ISH) have been employed for over 50 years.
These methods involve applying dyes or fluorescent probes to thin tissue sections mounted on microscope
slides to detect specific proteins and nucleic acids. IHC uses antibodies to identify the location of
proteins and other antigens within the tissue, while ISH targets specific DNA or RNA sequences. Fluorescence
microscopy enables the spatial mapping of gene expression, protein localization and me-
Credit: iStock
THE PAST, PRESENT AND FUTURE OF SPATIAL BIOLOGY 2
Listicle
tabolites using techniques like fluorescence ISH (FISH) and immunofluorescence (Figure 1).7 However,
these approaches have several limitations, including the need for prior knowledge of target molecules,
restricted multiplexing capabilities and challenges with spatial resolution.
Fixation Hybridization Fluorescence microscopy
presence of the target – triggers a signal that can be
visualized.
This method was first described in the 1960s and used
radiolabeling. Since then, ISH has been redefined using
different types of probes and methods to increase signal
intensity. Arguably the most well-known method being
fluorophore in situ hybridization (FISH).
In situ sequencing (ISS)
General concept: RNA is sequenced in a cell that remains in
its original tissue sample, preserving its morphology. The
first approach, published in 2013, utilized padlock probes that
target known RNA sequences.
FISH
ISS WITH PADLOCK PROBES
Reverse transcriptase creates cDNA of RNA target. Padlock
probe is introduced that can hybridize two regions of the
cDNA. Amplification of the target sequence is achieved via
rolling-circle amplification (RCA). RCA products are sequenced
by ligation, in situ.
Derivatives of the FISH method (developed
to overcome issues such as throughput,
autofluorescence background noise and
spectral overlaps) include but are not limited to:
Single molecule FISH (smFISH) — 2008
Sequential hybridization FISH (seqFISH) — 2014
Reverse transcription Hybridization Amplification Sequencing
In situ sequencing
In situ sequencing (ISS) refers to the targeted sequencing of RNA fragments within morphologically preserved
tissues or cells without RNA extraction (Figure 2). This method includes in situ cDNA synthesis by padlock
probes or stably cross-linked cDNA amplicons in fluorescent in situ RNA sequencing (FISSEQ) in addition to
in situ amplification by rolling-circle amplification (RCA).8,9 This technology allows for rapid gene expression
analysis within intact tissue samples at single-cell resolution, offering invaluable insights into complex biological
and pathological processes.
For example, in a recent study, ISS was used to explore the role of amyloid-beta plaques in Alzheimer’s
disease.10 The results showed that amyloid plaques trigger a strong, coordinated response among all cell
types, contributing to the surrounding inflammatory environment. ISS revealed transcriptional changes at the
single-cell level in both mouse and human brain sections, pinpointing early changes in oligodendrocyte-related
gene networks and later phase networks involving inflammation and oxidative stress. However, this
technique still requires prior knowledge of target genes and can struggle to accurately quantify gene expression
levels.
Figure 1: Fluorescent in situ hybridization (FISH). FISH involves hybridizing a labeled complementary fluorescent
probe to the target nucleic acid within the sample. After hybridization, any unbound probes are washed away and the
bound probes are visualized under a microscope. Credit: Technology Networks.
Figure 2: In situ sequencing (ISS) detects mRNA within tissues using padlock probes, which bind to target cDNA
sequences after mRNA is reverse transcribed and degraded. The probes are ligated to form circular DNA, which
is amplified by rolling circle amplification, producing localized rolling circle products (RCPs). These RCPs are then
sequenced using fluorescent probes to identify specific transcripts. Credit: Technology Networks.
Fixation Hybridization Fluorescence microscopy
In situ sequencing (ISS)
General concept: RNA is sequenced in a cell that remains in
its original tissue sample, preserving its morphology. The
first approach, published in 2013, utilized padlock probes that
target known RNA sequences.
ISS WITH PADLOCK PROBES
Reverse transcriptase creates cDNA of RNA target. Padlock
probe is introduced that can hybridize two regions of the
cDNA. Amplification of the target sequence is achieved via
rolling-circle amplification (RCA). RCA products are sequenced
by ligation, in situ.
More recently a number of variations of ISS have
been developed, using fluorescent probes and
cross-linking of a cell to its environment, and
barcode based-methods to improve amplification
efficiency and to allow for un-targeted studies.
Derivatives of the FISH method (developed
to overcome issues such as throughput,
autofluorescence background noise and
spectral overlaps) include but are not limited to:
Single molecule FISH (smFISH) — 2008
Sequential hybridization FISH (seqFISH) — 2014
Reverse transcription Hybridization Amplification Sequencing
THE PAST, PRESENT AND FUTURE OF SPATIAL BIOLOGY 3
Listicle
Imaging mass cytometry
Flow cytometry and mass spectrometry are essential tools for “omics” level measurements, enabling comprehensive
analysis of proteins on a large scale. First described in 2014, imaging mass cytometry (IMC)
integrates the principles of both flow cytometry and mass spectrometry to generate high-parameter images
of tissue sections.11
In IMC, metal-tagged antibodies bind to target proteins on the surface or within cells, and these proteins are
subsequently detected through mass spectrometry (Figure 3). The use of metal isotopes in IMC significantly
expands the number of proteins that can be targeted simultaneously compared to traditional flow cytometry.
This technique allows the simultaneous analysis of up to 40 markers in a single tissue section and is compatible
with both frozen and paraffin-embedded tissues.
A recent study utilized IMC to investigate the composition and spatial organization of immune and stromal
cells within the tumor microenvironments (TME) of advanced melanoma patients.12 By analyzing baseline
tumor samples from 26 patients receiving anti-programmed cell death-1 (anti-PD-1) therapy, the researchers
were able to conduct a detailed examination of both inter- and intra-tumor heterogeneity. This approach
led to the identification of six distinct TME archetypes based on their multicellular compositions. Notably, the
study revealed that patients with different TME archetypes exhibited varying responses to anti-PD-1 therapy,
highlighting the potential of IMC to inform personalized treatment strategies. However, this approach still has
disadvantages such as low resolution and time-consuming data acquisition.13
Spatial transcriptomics
First published in 2016, spatial transcriptomics (ST) uses unique positional barcodes to enable the unbiased
capture of RNA inside tissue sections.14 This technique combines traditional histological techniques with
high-throughput RNA sequencing to visualize and quantitatively analyze the entire transcriptome with spatial
distribution in tissue sections.
Figure 3: Imaging Mass Cytometry uses metal-tagged antibodies to label target proteins in tissue sections. A laser
ablates the tissue, releasing the metal tags, which are analyzed by mass spectrometry. The metal isotopes are
measured and mapped back to the tissue, creating highly multiplexed images that simultaneously reveal the spatial
distribution of multiple markers, enabling detailed analysis of tissue architecture and cell heterogeneity. Adapted
from a figure created by Schlecht A et al. Credit: Technology Networks.
UV-laser
mass cytometer
data analysis segmentation image processing image acqusition
marker staining laser ablation and mass cytometer
with metal-labeled
antibodies
THE PAST, PRESENT AND FUTURE OF SPATIAL BIOLOGY 4
Listicle
ST has rapidly evolved since it was first published, integrating various technologies to deliver high-resolution
gene expression mapping. ST technologies, including MERFISH and Slide-seq have transformed
our ability to map the transcriptome, providing high-throughput analysis with a spatial resolution of
sub-micrometer levels.15 These innovations led to ST being named “Method of the Year” by Nature
Methods in 2021.16 Currently, ST has extensive applications in various fields, such as cancer research,
developmental biology, pathology and organ chip technology.17,18,19,20
This field is continuing to expand and innovate, integrating cutting-edge technologies such as nanotechnology,
microfluidics and single-cell sequencing.
Spatial multi-omics technologies
The future of spatial biology lies in integrating spatial transcriptomics and proteomics with other omics
data to gain a deeper understanding of cellular functions within tissues. Spatial multi-omics has emerged
as a powerful method for comprehensive cell analysis in tissues, facilitating the parallel or even simultaneous
examination of multiple data types such as transcriptome, epigenome and proteome.
Advances in spatial multi-omics are rapidly evolving to allow the investigation of different molecular
analytes at subcellular resolution while preserving their native tissue context. Recognized by Nature as
one of the top technologies to watch in 2022, spatial multi-omics builds on established spatial techniques
such as IMC and ISS.21 These techniques can be applied on adjacent sections, serially on the
same section – if analyte quality remains intact – or in parallel on the same section when joint targeting
and analysis of multiple analytes are feasible.
For instance, techniques such as Spatial-CITE-seq (cellular indexing of transcriptomes and epitopes
by sequencing) and DBiT-seq (deterministic barcoding in tissue for spatial omics sequencing) combine
transcriptomics with proteomics.22,23 Additionally, methods like Spatial Cut&TAG and ATAC-RNAseq
have been developed to simultaneously analyze both epigenomics and transcriptomics, providing a
more comprehensive view of cellular processes.24 As this technology continues to develop, it’s likely
that even more processes will be combined for a comprehensive view of cellular function, allowing for
deeper insights into the interplay between gene expression, protein activity and chromatin dynamics
within the spatial context of tissues
Limitations and challenges
Spatial omics technologies face significant challenges, such as data complexity, computational demands
and the need for standardized analysis methods.25 One of the biggest hurdles lies in the sheer
complexity and scale of the data generated. Spatial omics data are often noisy, heterogeneous and influenced
by multiple biological and technical factors, including tissue preservation methods, sequencing
depth and sample preparation protocols. This makes it difficult to compare results across studies,
requiring the development of robust quality control and normalization procedures. The absence of
standardized pipelines for data analysis compounds these challenges, creating a barrier to the widespread
adoption of these techniques in clinical and research settings.26
While data acquisition is often seen as the most resource-intensive and technically demanding part of
spatial omics experiments, the downstream processes of data manipulation, analysis and visualization
are equally crucial. Advanced computational tools, including image analysis algorithms, dimensionality
reduction techniques and network-based approaches, are essential for processing and interpreting this
data. Without the right tools, even well-designed and expensive experiments can result in misleading
or incomplete conclusions, wasting time and resources.
THE PAST, PRESENT AND FUTURE OF SPATIAL BIOLOGY 5
Listicle
To address these challenges and push the field forward, several key developments are needed:
• Enhancing spatial resolution to achieve subcellular detail
• Expanding molecular coverage, throughput and multiplexing capabilities
• Developing standardized, integrated experimental and computational methods for multi-modal spatial
data analysis
• Creating comprehensive atlases that span entire organisms, mapping cellular and molecular features
across tissues and systems
The future of spatial biology
Spatial biology represents a groundbreaking fusion of molecular biology and advanced imaging tools, transforming
our understanding of cellular organization and function in tissues. It provides unparalleled insights into
genes, proteins and RNA within a tissue’s context, reshaping research, diagnostics and therapeutic strategies.
While challenges remain in data complexity, computational tools and standardization, advancements in spatial
omics technologies are rapidly overcoming these barriers. As the field continues to evolve, it holds the promise
of more precise and personalized medicine, driving progress in areas like cancer research, neurodegenerative
diseases and drug discovery.
Spatial biology is not just the future of biomedical research – it is the key to unlocking the full complexity of life.
References:
1. Locke D, Hoyt CC. Companion diagnostic requirements for spatial biology using multiplex immunofluorescence and multispectral
imaging. Front Mol Biosci. 2023;10. doi: 10.3389/fmolb.2023.1051491
2. Cheng M, Jiang Y, Xu J, et al. Spatially resolved transcriptomics: a comprehensive review of their technological advances,
applications, and challenges. J Genet Genom. 2023;50(9):625-640. doi: 10.1016/j.jgg.2023.03.011
3. Zhang X, Wang X, Shivashankar GV, Uhler C. Graph-based autoencoder integrates spatial transcriptomics with chromatin
images and identifies joint biomarkers for Alzheimer’s disease. Nat Commun. 2022;13(1):7480. doi: 10.1038/s41467-022-
35233-1
4. Fu F, Nowak MA, Bonhoeffer S. Spatial heterogeneity in drug concentrations can facilitate the emergence of resistance to
cancer therapy. PLoS Comput Biol. 2015;11(3):e1004142. doi: 10.1371/journal.pcbi.1004142
5. Dominiak A, Chełstowska B, Olejarz W, Nowicka G. Communication in the cancer microenvironment as a target for therapeutic
interventions. Cancers. 2020;12(5):1232. doi: 10.3390/cancers12051232
6. Sharma P, Aaroe A, Liang J, Puduvalli VK. Tumor microenvironment in glioblastoma: Current and emerging concepts.
Neuro-oncol adv. 2023;5(1):vdad009. doi: 10.1093/noajnl/vdad009
7. Alexandrov T, Saez‐Rodriguez J, Saka SK. Enablers and challenges of spatial omics, a melting pot of technologies. Mol
Syst Biol. 2023;19(11):e10571. doi: 10.15252/msb.202110571
8. Ke R, Mignardi M, Pacureanu A, et al. In situ sequencing for RNA analysis in preserved tissue and cells. Nat Methods.
2013;10(9):857-860. doi: 10.1038/nmeth.2563
9. Lee JH, Daugharthy ER, Scheiman J, et al. Highly multiplexed subcellular RNA sequencing in situ. Science.
2014;343(6177):1360-1363. doi: 10.1126/science.1250212
10. Chen WT, Lu A, Craessaerts K, et al. Spatial transcriptomics and in situ sequencing to study Alzheimer’s disease. Cell.
2020;182(4):976-991.e19. doi: 10.1016/j.cell.2020.06.038
11. Giesen C, Wang HAO, Schapiro D, et al. Highly multiplexed imaging of tumor tissues with subcellular resolution by mass
cytometry. Nat Methods. 2014;11(4):417-422. doi: 10.1038/nmeth.2869
12. Xiao X, Guo Q, Cui C, et al. Multiplexed imaging mass cytometry reveals distinct tumor-immune microenvironments
linked to immunotherapy responses in melanoma. Commun Med. 2022;2(1):1-14. doi: 10.1038/s43856-022-00197-2
13. Milosevic V. Different approaches to Imaging Mass Cytometry data analysis. Bioinformatics Advances. 2023;3(1):vbad046.
doi: 10.1093/bioadv/vbad046
THE PAST, PRESENT AND FUTURE OF SPATIAL BIOLOGY 6
Listicle
14. Ståhl PL, Salmén F, Vickovic S, et al. Visualization and analysis of gene expression in tissue sections by spatial transcriptomics.
Science. 2016;353(6294):78-82. doi: 10.1126/science.aaf2403
15. Moses L, Pachter L. Museum of spatial transcriptomics. Nat Methods. 2022;19(5):534-546. doi: 10.1038/s41592-022-01409-2
16. Marx V. Method of the Year: spatially resolved transcriptomics. Nat Methods. 2021;18(1):9-14. doi: 10.1038/s41592-020-
01033-y
17. Arora R, Cao C, Kumar M, et al. Spatial transcriptomics reveals distinct and conserved tumor core and edge architectures
that predict survival and targeted therapy response. Nat Commun. 2023;14(1):5029. doi: 10.1038/s41467-023-40271-4
18. Choe K, Pak U, Pang Y, Hao W, Yang X. Advances and challenges in spatial transcriptomics for developmental biology.
Biomolecules. 2023;13(1):156. doi: 10.3390/biom13010156
19. Pang JMB, Byrne DJ, Bergin ART, et al. Spatial transcriptomics and the anatomical pathologist: Molecular meets morphology.
Histopathology. 2024;84(4):577-586. doi: 10.1111/his.15093
20. Li D, Fang Z, Shi Q, et al. Single-cell RNA-sequencing and subcellular spatial transcriptomics facilitate the translation
of liver microphysiological systems for regulatory application. J Pharm Anal. 2023;13(7):691-693. doi: 10.1016/j.
jpha.2023.06.013
21. Eisenstein M. Seven technologies to watch in 2022. Nature. 2022;601(7894):658-661. doi: 10.1038/d41586-022-00163-x
22. Liu Y, DiStasio M, Su G, et al. High-plex protein and whole transcriptome co-mapping at cellular resolution with spatial
CITE-seq. Nat Biotechnol. 2023;41(10):1405-1409. doi: 10.1038/s41587-023-01676-0
23. Liu Y, Yang M, Deng Y, et al. High-spatial-resolution multi-omics sequencing via deterministic barcoding in tissue. Cell.
2020;183(6):1665-1681.e18. doi: 10.1016/j.cell.2020.10.026
24. Li X. Harnessing the potential of spatial multiomics: a timely opportunity. Sig Transduct Target Ther. 2023;8(1):1-3. doi:
10.1038/s41392-023-01507-3
25. Mulholland E, Leedham S. Redefining clinical practice through spatial profiling: a revolution in tissue analysis. annals.
2024;106(4):305-312. doi: 10.1308/rcsann.2023.0091
26. Williams CG, Lee HJ, Asatsuma T, Vento-Tormo R, Haque A. An introduction to spatial transcriptomics for biomedical
research. Genome Med. 2022;14(1):68. doi: 10.1186/s13073-022-01075-1
About the author:
Bree Foster, PhD, is a Science Writer for Technology Networks
Sponsored by: